Physiology of Endurance Training
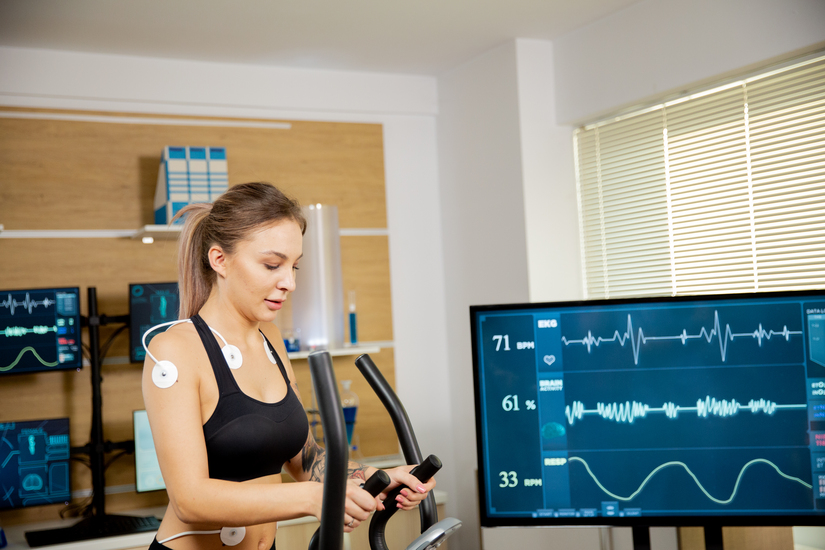
Endurance Training
The primary anatomical components of the cardiopulmonary system encompass the lungs, heart, and skeletal muscles. In the capillary beds of the lungs, blood unloads carbon dioxide (CO2) and takes up oxygen (O2). This oxygen-enriched blood proceeds from the lungs to the heart through the pulmonary vein. It first enters the left atrium and then flows into the left ventricle. Upon the heart’s contraction, oxygen-enriched blood is propelled from the left ventricle and exits the heart through the aorta. The aorta subsequently branches into smaller arteries, which distribute oxygen-enriched blood throughout the entire body.
As the oxygen-enriched blood reaches various parts of the body, such as the leg muscles during running, it unloads oxygen and acquires carbon dioxide. Blood leaving the exercising muscles is now “oxygen reduced” and returns to the heart via the venous system. This oxygen-reduced blood is ultimately delivered to the heart via two major veins, the superior and inferior vena cava. These vessels introduce oxygen-reduced blood into the right atrium of the heart, which then flows into the right ventricle. With the heart’s contraction, oxygen-reduced blood is ejected from the right ventricle and travels through the pulmonary artery back to the lungs.
And thus, we have come full circle in our tour of cardiopulmonary anatomy. As the oxygen-reduced blood enters the capillary beds of the lungs, it releases carbon dioxide and picks up oxygen, emerging from the lungs as oxygen-enriched blood. This seamless coordination among the lungs, heart, and body tissues occurs continually, whether the individual is awake or asleep. The entire cardiopulmonary system operates diligently during any endurance-based sporting activity, such as a triathlon.
Oxygen Transport
Endurance sports place a significant reliance on the oxidative phosphorylation energy system, which is intricately linked to oxygen availability. In the previous section, we discussed oxygen transport in general terms, referring to oxygen-enriched and oxygen-reduced blood. In this section, we’ll delve into the specifics of oxygen transport, focusing on the gas physics and physiology of this vital process.
To understand oxygen transport, it’s essential to consider how oxygen moves within the body. Although a small portion of oxygen dissolves in the fluid component of the blood, the primary mode of oxygen transport throughout the body is through red blood cells, also known as erythrocytes. These red blood cells are incredibly numerous, with trillions present in the bloodstream. The part of blood that contains red blood cells is termed the hematocrit (Hct), expressed as a percentage relative to the total blood volume. In healthy individuals residing at low elevations, hematocrit values typically range from 35 to 45 percent for women and 40 to 50 percent for men.
Each red blood cell contains around 250 million molecules of hemoglobin (Hb), the crucial molecule responsible for oxygen transportation. A single hemoglobin molecule has the capacity to transport four oxygen molecules. Consequently, a single red blood cell can carry approximately 1 billion oxygen molecules, and the human body contains trillions of these red blood cells.
Now that we comprehend that oxygen transport primarily occurs through red blood cells, specifically via the hemoglobin molecules within these cells, let’s explore how oxygen-reduced blood becomes oxygen-enriched blood in the lungs. This entire process of oxygen transport is precisely regulated by changes in the partial pressure of oxygen (PO2) from the moment we inhale air through our nose and mouth to the point where the air reaches the body’s tissues and organs. PO2 gradually decreases as inspired air travels from the nose and mouth to the lungs, driven by the process of diffusion, where molecules move from areas of high concentration to areas of low concentration. At sea level, the PO2 of inspired air is roughly 159 mm Hg, which drops to 105 mm Hg in the lungs.
Blood entering the lungs through the pulmonary arteries carries red blood cells relatively low in oxygen. The PO2 of this oxygen-reduced blood is approximately 40 mm Hg. The pressure gradient in the lungs (105 mm Hg) versus the oxygen-reduced blood (40 mm Hg) favors the diffusion of oxygen from the lungs to the blood. This oxygen then binds to hemoglobin molecules. The diffusion of oxygen in the lungs takes about 0.75 seconds and occurs across an incredibly thin membrane in the pulmonary capillaries, which is about 1/10,000 the thickness of a Kleenex tissue. As a result of this diffusion, oxygen-enriched blood exits the lungs with a PO2 of 100 mm Hg. This oxygen-enriched blood is transported by pulmonary veins to the left ventricle of the heart, and from there, it is circulated throughout the body, as previously explained. When this oxygen-enriched blood reaches the capillary beds of a skeletal muscle, the pressure gradient favors the release of oxygen from hemoglobin to the muscle. The oxygen-enriched blood has a PO2 of around 100 mm Hg, while the muscle maintains a PO2 of approximately 30 mm Hg. The released oxygen in the skeletal muscle is now available for the mitochondria to use in the production of ATP through the oxidative phosphorylation energy system. Subsequently, the blood exits the capillary bed of the muscle, now oxygen-reduced with a PO2 of approximately 40 mm Hg. This oxygen-reduced blood returns to the right ventricle of the heart, and the process of oxygenation in the lungs and oxygen transport throughout the body repeats.
The efficiency of oxygen transport is a pivotal factor in optimizing performance in endurance sports, primarily those reliant on oxidative energy production. Athletes often wonder if it’s possible to enhance their cardiopulmonary system and oxygen transport capabilities through training, and the answer is unequivocally yes.
One approach to enhancing oxygen transport involves altitude training. This training method leads to an increase in the number of red blood cells and hemoglobin molecules, subsequently augmenting the capacity to deliver oxygen to the working muscles. Nevertheless, not all athletes have the luxury of time or resources to undertake prolonged altitude training that’s required to yield these physiological adaptations. Unfortunately, some unethical athletes resort to illegal pharmacological ergogenic aids, such as recombinant human erythropoietin (rhEPO), to induce similar effects. It’s vital for athletes to comprehend that several positive cardiopulmonary training effects can be achieved at sea level by adhering to a well-designed and scientifically grounded training regimen.
This section zeroes in on these advantageous cardiopulmonary training effects. Numerous cardiopulmonary adaptations transpire due to regular endurance training, which necessitates a minimum of 30 to 45 minutes per training session and a minimum of 3 to 5 training sessions each week for at least 8 weeks. These adaptations encompass the following:
- A reduction in resting and exercise heart rate.
- An increase in total blood volume.
- An elevation in cardiac output.
- Augmented exercise respiratory capacity.
- An increase in maximal oxygen uptake (VO2max).
- Enhancement of the lactate threshold.
- Improvement in maximal exercise performance.
- Improvement in exercise economy.
- Improvement in endurance performance.
- Enhancement in heat tolerance.
- A decrease in total body weight.
- A decrease in body fat.
- A decrease in blood pressure (if moderate or high blood pressure is present).
The cumulative effects of training-induced improvements in cardiac output, VO2max, lactate threshold, exercise economy, and maximal exercise performance undeniably have a favorable impact on endurance performance. After all, performance enhancement is the ultimate goal for every endurance athlete and coach. However, substantial improvements in endurance performance will not occur unless the necessary training is undertaken to provoke these advantageous cardiopulmonary training effects. The forthcoming sections delve into some of these benefits in greater detail.
Heart Rate
Regular endurance training triggers a process of progressive overload, which leads to the strengthening of the heart. A stronger heart is capable of pumping out more blood with each beat, thereby reducing the effort it has to exert. Consequently, an individual’s heart rate at rest and during exercise will decrease compared to their pre-training values. During exercise, the heart rate at a given workload will also be lower. For instance, if someone’s heart rate was 175 beats per minute (bpm) after running 800 meters on the first day of training, after 8 weeks of endurance training, that heart rate should significantly decrease when running the same 800 meters at the same pace.
Endurance training also enhances a person’s recovery heart rate. Using the same example, if it initially took 3 minutes for a person’s heart rate to drop from 175 bpm to 125 bpm after running 800 meters, after 8 weeks of training, the recovery heart rate should improve significantly, requiring less than 3 minutes to return from 175 bpm to 125 bpm. Although the magnitude of improvement varies among individuals, it is safe to expect that after a minimum of 8 weeks of endurance training, improvements in resting heart rate (lower), exercise heart rate at the same workload (lower), and recovery heart rate (less time to recover) will manifest.
Cardiac Output
Endurance training contributes to an increase in specific hormones that regulate blood volume. These hormones work to elevate the fluid portion of the blood, known as plasma. The net result of this hormonal response is a rise in total blood volume. With a stronger and more efficient heart, this greater blood volume means that the heart can pump more blood over a specific timeframe (at the same heart rate). This increase in the quantity of blood pumped over a given period is known as an augmentation in cardiac output. Cardiac output quantifies the volume of blood the heart pumps through the body in one minute. An increased cardiac output is essential because it results in more blood being delivered to vital organs like the brain, liver, kidneys, and, notably, to the working skeletal muscles during endurance exercise. Consequently, this facilitates the delivery of more oxygen to the active muscles for energy production while concurrently hastening the removal of carbon dioxide and other metabolic by-products from the exercising muscles.
VO2max
Endurance training also enhances the lung capacity during exercise. This means that the individual’s respiratory rate (number of breaths per minute) and tidal volume (volume of air per breath) improve. These improvements in lung capacity can contribute to an elevation in maximal oxygen uptake (VO2max). Maximal oxygen uptake is the highest volume of oxygen a person’s body can consume and utilize for aerobic energy production. VO2max can be expressed either in absolute units (liters of oxygen per minute [L × min^-1]) or relative units (milliliters of oxygen per kilogram of body mass per minute [ml × kg^-1 × min^-1]). VO2max is generally expressed in relative units (ml × kg^-1 × min^-1) as it allows for comparisons between individuals, indicating who is the fittest concerning their body weight.
Well-trained female and male endurance athletes can reach VO2max levels of 65 to 75 ml × kg^-1 × min^-1 and 75 to 85 ml × kg^-1 × min^-1, respectively. In contrast, untrained females and males usually have values that range from 35 to 40 ml × kg^-1 × min^-1 and 45 to 50 ml × kg^-1 × min^-1, respectively. An improvement in VO2max is of utmost importance as it signifies that more oxygen is accessible to the working muscles for energy production. Scientific research has established that a high VO2max is one of the key physiological factors contributing to success in endurance sports such as distance running, cross-country skiing, and triathlon.
Lactate Threshold and Maximal Exercise Performance
Recent scientific research has identified two additional crucial physiological factors that play a significant role in endurance performance: lactate threshold (LT) and maximal exercise performance. These physiological parameters are typically assessed in a controlled laboratory setting.
Lactate threshold denotes the point in an escalating endurance training session or race at which the athlete’s body necessitates a greater contribution from the glycolysis energy system (short-term energy system) and a lesser contribution from the oxidative phosphorylation energy system (long-term energy system). This leads to a scenario where lactate production surpasses lactate removal, resulting in a rapid increase in blood lactate levels. Therefore, a higher lactate threshold is advantageous for endurance performance. In the context of triathletes, swimming velocity (m × sec^-1), cycling power output (watts per kilogram of body weight [W × kg^-1]), and running velocity (m × min^-1) are the key measurements of interest. Participation in a well-structured endurance training program is likely to yield substantial improvements in these lactate threshold parameters. Athletes can anticipate improvements within a single season and, depending on their training history, from season to season.
Maximal exercise performance serves as a quantification of an endurance athlete’s athletic capacity, gauged at the point when the athlete voluntarily terminates exercise due to exhaustion (volitional exhaustion). This is determined at the conclusion of a laboratory-based maximal exercise test, such as a treadmill test. The same physiological measurements employed for assessing lactate threshold are pertinent here, but they are assessed under conditions of maximal effort instead of lactate threshold effort. Like the lactate threshold, higher maximal exercise performance is conducive to better endurance performance. By engaging in a well-structured endurance training program, athletes can expect the same type of improvement in maximal exercise performance as seen with the lactate threshold, both within a single season and from season to season.
Physiological Economy
In the context of endurance performance, one vital physiological factor to consider is economy. This concept can be likened to fuel efficiency in an automobile. Just as a more economical or efficient car utilizes less gasoline at a particular speed and achieves superior “miles per gallon” than a less economical vehicle, the same principles apply to endurance athletes.
Let’s illustrate this with a hypothetical scenario: Athlete A and Athlete B both possess similar V˙ O2max values of 65 ml × kg^-1 × min^-1. However, during the first half of a 10K race when they are running at a pace of 5 minutes per mile, Athlete A expends only 50 ml × kg^-1 × min^-1, whereas Athlete B consumes 53 ml × kg^-1 × min^-1 at the same pace. Consequently, Athlete A is more efficient and economical in terms of energy expenditure because they utilize less oxygen, akin to a vehicle using less gas. This enhanced physiological economy positions Athlete A at a competitive advantage over Athlete B, particularly in the latter stages of the race.
Various factors contribute to improving physiological economy, including a well-designed endurance training program, individual running biomechanics, uphill running, bungee running, and plyometrics training. In the realm of endurance competitions, a heightened physiological economy becomes pivotal for achieving success.
Tolerance to Heat and Humidity
Endurance training significantly enhances an individual’s ability to work and exercise in hot and humid conditions. As previously mentioned, consistent endurance training results in an increase in plasma production and total blood volume. Think of total blood volume as the equivalent of radiator coolant in a vehicle. By increasing total blood volume, endurance training equips the body with additional “radiator coolant,” enabling the individual to produce more sweat and dissipate heat more effectively, particularly when engaged in physical activities in hot and humid environments. This has particularly valuable implications for endurance athletes who frequently compete in arid or tropical climates.
Body Weight and Body Fat
Endurance training is conducive to reducing a person’s total body weight and decreasing body fat. This might not be a primary concern for well-trained endurance athletes, as they tend to maintain lean physiques. Nevertheless, it becomes more pertinent as athletes age, particularly when their lifestyles, such as work, family, or travel commitments, hinder them from training as rigorously as in their earlier career stages. For individuals with moderate to high blood pressure, regular endurance training can yield a significant reduction, thus diminishing the risk of cardiovascular disease and premature mortality. This is usually less pertinent for well-trained endurance athletes since they typically have normal blood pressure. However, elevated blood pressure may emerge as an issue as athletes age and their activity levels decline.
Training Effects on Skeletal Muscle
Skeletal muscle can be modified in response to different types of training, specifically aerobic (endurance) training, anaerobic (sprint) training, and resistance training. Each type of training leads to specific physiological and biochemical adaptations in skeletal muscle. Let’s delve into how each type of training affects skeletal muscle.
Aerobic or Endurance Training:
Endurance training primarily targets Type I (slow oxidative) muscle fibers over Type IIX (fast glycolytic) muscle fibers, leading to specific changes in skeletal muscle composition. Although the percentage of Type I and Type IIX fibers typically remains unchanged, endurance training may cause Type IIX fibers to adopt characteristics similar to Type IIA (fast oxidative glycolytic) fibers when regularly recruited during exercise.
A key effect of endurance training is the increase in the number of capillaries supplying each muscle fiber. These tiny, meshlike structures embedded deep in the muscle enhance the delivery of oxygen and nutrients while facilitating the removal of metabolic by-products like carbon dioxide and lactate. This increased capillary density enables more efficient muscle function.
Endurance training also results in the growth of both the number and size of mitochondria within skeletal muscle. This effect is particularly notable in Type I (slow oxidative) muscle fibers. Mitochondria are essential for ATP production through the oxidative phosphorylation energy system, thereby enhancing oxidative energy production.
The activity of many oxidative enzymes is boosted with endurance training. These enzymes are primarily involved in the Krebs cycle phase of the oxidative phosphorylation energy system. Enhanced enzymatic activity further supports oxidative energy production, contributing to better endurance and performance.
Lastly, endurance training elevates muscle myoglobin content by approximately 75 to 80 percent. Myoglobin, similar in structure to hemoglobin, transports oxygen within the muscle. While hemoglobin carries oxygen to muscles through the bloodstream, myoglobin picks up oxygen in the capillary bed and transports it to the mitochondria for ATP synthesis. Increased myoglobin content enhances oxygen delivery to working muscles during exercise.
Anaerobic or Sprint Training:
Anaerobic training leads to specific adaptations within skeletal muscle that cater to the requirements of high-intensity, short-duration activities. These adaptations include an increase in the activity of ATP-CP and glycolytic enzymes, many of which are involved in the immediate and short-term energy systems for ATP production. This heightened enzymatic activity enhances the efficiency of these energy systems, allowing for rapid ATP generation during intense exercise.
One notable benefit of anaerobic training is the enhancement of skeletal muscle buffering capacity. This capacity is vital for neutralizing the accumulation of lactate and positively charged hydrogen ions (H+) produced in the glycolysis energy system. High levels of H+ can hinder the release of calcium (Ca2+) during the excitation phase of muscle contraction, potentially leading to muscle fatigue. Anaerobic training increases bicarbonate (HCO3–) content within skeletal muscle, which acts as a highly effective buffer, countering acidosis by neutralizing H+ ions and safely removing them from the body as water (H2O) and carbon dioxide (CO2).
By increasing buffering capacity, anaerobic training helps delay the onset of muscle fatigue during high-intensity efforts, allowing athletes to perform at their best for short durations.
These physiological adaptations in skeletal muscle demonstrate its remarkable ability to adjust to various training demands, supporting optimal performance in endurance, sprint, and resistance activities.