Adaptations to Resistance Training
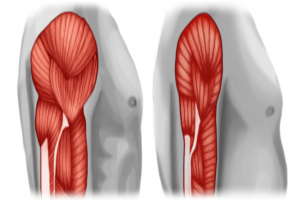
Neuromuscular Adaptations to Chronic Exercise:
Chronic exercise leads to a range of adaptations in the neuromuscular system. The extent of these adaptations depends on the type of training program undertaken. Aerobic training, like jogging or swimming, primarily targets cardiovascular fitness and endurance and typically results in little to no significant gain in muscular strength and power. In contrast, resistance training triggers major neuromuscular adaptations.
In the past, resistance training was often considered suitable only for specific athletes, such as those in competitive weightlifting, track and field weight events, football, wrestling, and boxing. There were even restrictions on women’s access to weight rooms. However, a significant shift in perspective occurred in the late 1960s and early 1970s when coaches and researchers recognized the benefits of strength and power training for a wide range of sports and activities, including women. Moreover, in the late 1980s and early 1990s, health professionals began to acknowledge the importance of resistance training for overall health and fitness.
Today, most athletes incorporate strength and power training as crucial components of their training regimens. This change in attitude towards resistance training can be attributed to research findings that demonstrate its performance benefits, as well as innovations in training techniques and equipment. Resistance training is now a fundamental aspect of exercise prescriptions for individuals seeking health-related benefits from their physical activity.
Resistance Training and Gains in Muscular Fitness:
Muscular fitness plays a pivotal role in athletic performance and overall health. Resistance training programs are essential for increasing muscular strength, power, and endurance. While maintaining an active lifestyle is crucial for preserving muscular fitness, resistance training is necessary to achieve significant improvements in these areas.
This section provides an overview of the changes that result from resistance training, with a focus on strength, although it briefly mentions power and muscular endurance. The details about power and muscular endurance are covered more extensively in later sections of this book.
The neuromuscular system is particularly responsive to resistance training. Such programs can yield substantial gains in strength within three to six months. These improvements can range from 25% to 100% or even more. However, it’s important to recognize that percentage gains can be somewhat misleading. Many individuals who participate in strength training research studies have never engaged in resistance training before. A significant portion of their initial strength gains is attributed to learning how to more effectively generate force and perform maximal movements. For example, individuals learn how to lift a barbell from their chest to a fully extended position in the bench press. This learning effect can contribute to as much as 50% of the overall strength gain.
Notably, gains in strength tend to be similar across different populations, regardless of gender, age, or fitness level when expressed as a percentage of their initial strength. However, the absolute increase in the weight lifted may differ. For instance, after 20 weeks of resistance training, assume that a 12-year-old boy and a 25-year-old man each improve their bench press strength by 50%. If the man’s initial bench press strength (one-repetition maximum, 1RM) was 50 kg (110 lb), he would have enhanced his strength by 25 kg (55 lb), reaching a new 1RM of 75 kg (165 lb). In contrast, the boy’s initial 1RM was 25 kg, so his improvement would be 12.5 kg (28 lb), resulting in a new 1RM of 37.5 kg (83 lb).
Muscle exhibits plasticity, meaning it can increase in size and strength with exercise training and decrease in size and strength when subjected to immobilization. The remainder of this section delves into the physiological adaptations that occur to enable individuals to become stronger. It also addresses the acute muscle pain experienced in the specific muscles trained during the initial weeks of resistance training.
Mechanisms of Muscle Strength Gains:
For many years, it was commonly believed that gains in muscle strength were primarily a result of increases in muscle size, or hypertrophy. This notion seemed logical because individuals who engaged in regular strength training often developed visibly larger muscles. Conversely, when muscles were immobilized in a cast for extended periods, they rapidly decreased in size (atrophy) and lost strength. There appeared to be a direct association between muscle size and strength, with gains in muscle size typically paralleling gains in strength, and conversely, losses in muscle size closely correlating with decreases in strength. However, muscle strength involves more complex mechanisms than just muscle size.
This is not to say that muscle size is insignificant in determining muscle strength. In fact, muscle size is a crucial factor, as evidenced by the world records for competitive weightlifting, where as weight classifications increase (indicating greater muscle mass), the total weight lifted also increases. Nevertheless, the mechanisms governing strength gains are intricate and not fully comprehended. Beyond muscle size, what explains the strength gains that result from resistance training? Emerging evidence suggests that alterations in the neural control of the trained muscle play a significant role in enabling greater force production.
Neural Control of Strength Gains:
An essential neural component contributes to at least some of the strength gains arising from resistance training. Enoka, in particular, has argued persuasively that strength gains can occur without structural changes in muscle but not without neural adaptations. This perspective highlights that strength is not solely a property of muscle tissue but rather a property of the motor system. Factors related to motor unit recruitment, stimulation frequency, and other neural elements are also critical in achieving strength gains. These neural adaptations may account for most, if not all, of the strength gains observed in the absence of muscle hypertrophy, and they could potentially explain extraordinary feats of strength.
Synchronization and Recruitment of Additional Motor Units:
Motor units are typically recruited asynchronously, meaning that not all motor units are activated simultaneously. Various neurons control motor units and transmit either excitatory or inhibitory impulses. The muscle fibers of a motor unit contract only when the incoming excitatory impulses exceed the inhibitory impulses, meeting or surpassing the threshold for activation.
Strength gains may occur due to changes in the connections between motor neurons within the spinal cord. These adaptations could enable motor units to act more synchronously, promoting muscle contraction and enhancing the muscle’s capacity to generate force. While evidence supports increased motor unit synchronization resulting from resistance training, there is still some debate about whether synchronization leads to more forceful contractions. Nonetheless, it is clear that synchronization improves the rate of force development and the ability to exert steady forces.
Another possibility is that more motor units are recruited for a given task, regardless of whether they act synchronously. This improvement in motor unit recruitment patterns could result from an increase in neural drive to alpha-motor neurons during maximal contractions. This heightened neural drive might also increase the frequency of motor unit discharge (rate coding) or reduce inhibitory impulses, allowing more motor units to be activated or activated at a higher frequency. These neural adaptations contribute significantly to the overall strength gains observed with resistance training.
Increased Rate Coding of Motor Units:
Enhanced neural drive to alpha-motor neurons resulting from resistance training can also lead to an increase in the frequency of discharge, known as rate coding, in their corresponding motor units. Recall from earlier discussions that when the frequency of stimulation of a motor unit is elevated, the muscle eventually enters a state of tetanus, reaching its maximum force or tension production. While there is limited evidence supporting an increase in rate coding with resistance training, it appears that rapid movements or ballistic-type training can be particularly effective in stimulating these increases.
Autogenic Inhibition:
Within the neuromuscular system, inhibitory mechanisms, such as the Golgi tendon organs, serve to prevent muscles from exerting more force than the bones and connective tissues can safely handle. This control mechanism is known as autogenic inhibition. However, in extreme situations where larger forces are occasionally generated, significant damage can occur to these structures, suggesting that protective inhibitory mechanisms can be overridden.
Golgi tendon organs, become activated when the tension on a muscle’s tendons and internal connective tissue structures exceeds a certain threshold. In response, motor neurons to that muscle are inhibited, resulting in autogenic inhibition. Both the reticular formation in the brain stem and the cerebral cortex play roles in initiating and propagating these inhibitory impulses.
Through training, these inhibitory impulses can gradually diminish or be counteracted, enabling the muscle to achieve greater levels of strength. Therefore, strength gains may partially arise from the reduction of neurological inhibition. This theory is attractive because it can help explain extraordinary displays of strength and strength gains even in the absence of muscle hypertrophy.
Other Neural Factors:
In addition to increased motor unit recruitment and reduced neurological inhibition, several other neural factors can contribute to strength gains through resistance training. One such factor is the coactivation of agonist and antagonist muscles. The agonist muscles are responsible for the primary movement, while the antagonist muscles work to impede the agonists. For instance, in forearm flexor concentric contractions, the biceps function as the primary agonist, and the triceps act as the antagonist. If both contract with equal force development, no movement would occur. Therefore, to maximize the force generated by an agonist, minimizing coactivation is necessary. A reduction in coactivation might explain some of the strength gains attributed to neural factors, although its contribution is likely relatively minor.
Additionally, changes in the morphology of the neuromuscular junction have been observed, featuring both increased and decreased activity levels. These changes may be directly related to the muscle’s capacity to produce force.
Muscle Hypertrophy:
Muscle hypertrophy is the process of increasing a muscle’s size, and it can occur in two forms: transient hypertrophy and chronic hypertrophy.
Transient Hypertrophy:
Transient hypertrophy refers to the temporary increase in muscle size that occurs during and immediately after a single exercise session. This increase is primarily due to fluid accumulation (edema) within the muscle’s interstitial and intracellular spaces, which originates from blood plasma. Transient hypertrophy is, as the name suggests, short-lived and typically lasts only for a brief period. The fluid accumulated during exercise returns to the bloodstream within hours after the exercise session.
Chronic Hypertrophy:
Chronic hypertrophy is characterized by the long-term increase in muscle size resulting from sustained resistance training over an extended period. Unlike transient hypertrophy, chronic hypertrophy reflects actual structural changes within the muscle. These changes can include an increase in the size of existing individual muscle fibers (fiber hypertrophy), an increase in the number of muscle fibers (fiber hyperplasia), or a combination of both. The exact mechanisms underlying chronic hypertrophy are a topic of ongoing debate and research.
It is worth noting that the eccentric component of resistance training (muscle lengthening during contraction) plays a crucial role in maximizing muscle fiber cross-sectional area. Studies have shown that eccentric contraction training alone can lead to greater hypertrophy and strength gains compared to concentric contraction training or a combination of both. Additionally, high-velocity eccentric training appears to result in more substantial hypertrophy and strength improvements than slower-velocity training. These increased gains are thought to be related to disruptions in the sarcomere Z-lines, which were originally labeled as muscle damage but are now considered a part of fiber protein remodeling. Therefore, resistance training involving only concentric actions may limit muscle hypertrophy and strength gains.
Fiber Hypertrophy:
Fiber hypertrophy is one mechanism contributing to increased muscle size through resistance training. Early research suggested that the number of muscle fibers within an individual’s muscles is established either at birth or shortly thereafter, remaining fixed throughout life. If this were true, muscle hypertrophy could occur only through individual muscle fiber hypertrophy. This increase in fiber size can be attributed to several factors, including:
- More myofibrils within each muscle fiber.
- More actin and myosin filaments within each muscle fiber.
- Increased sarcoplasm (the fluid within the muscle fiber).
- Enhanced connective tissue growth.
Fiber hypertrophy typically involves an increase in muscle protein synthesis, as the muscle’s protein content is in a constant state of flux. During exercise, protein synthesis decreases while protein degradation increases. However, during the post-exercise recovery period, the balance shifts toward a net protein synthesis. Consuming a carbohydrate and protein supplement immediately after a training session can promote a more positive nitrogen balance, facilitating protein synthesis and maximizing the muscle’s adaptive response to resistance exercise.
The hormone testosterone is believed to play a significant role in muscle hypertrophy, as one of its primary functions is to promote muscle growth. For instance, males experience a substantial increase in muscle growth during puberty due to a tenfold rise in testosterone production. Testosterone is a steroidal hormone with prominent anabolic (muscle-building) functions. It is well-established that large doses of anabolic steroids, when combined with resistance training, can markedly increase muscle mass and strength.
Fiber Hyperplasia:
Fiber hyperplasia is a potential mechanism contributing to muscle hypertrophy, involving an increase in the number of muscle fibers. Research conducted on animals, particularly cats, has provided insights into the potential occurrence of fiber hyperplasia in muscle hypertrophy.
Evidence from Animal Studies:
- Cat Studies: Cats were subjected to intense strength training, motivating them to generate significant force by moving heavy weights with their forepaws. With this rigorous strength training, certain muscle fibers in the cats appeared to split in half, with each half subsequently increasing in size to match the parent fiber. Cross-sectional cuts through the muscle fibers supported this observation.
- Subsequent Studies in Other Animals: Studies involving chickens, rats, and mice suggested that muscle hypertrophy resulting from chronic exercise overload was mainly due to the hypertrophy of existing muscle fibers, not fiber hyperplasia. Direct fiber counts in these studies revealed no change in fiber number.
Cat Study Revisited: Scientists revisited the initial cat experiments, conducting resistance training with cats and directly counting muscle fibers to determine if total muscle hypertrophy resulted from hyperplasia or fiber hypertrophy. After 101 weeks of resistance training, the cats demonstrated an 11% increase in muscle weight and a 9% increase in the total number of muscle fibers, confirming the occurrence of muscle fiber hyperplasia in response to resistance training in cats.
Training Differences: Differences in training methods likely explain the disparities between the cat studies and those involving rats and mice. Cats underwent pure resistance training characterized by high resistance and low repetitions, while other animals engaged in more endurance-type activities with low resistance and high repetitions.
Human Muscle Hypertrophy: While animal studies suggest the possibility of fiber hyperplasia, its role in human muscle hypertrophy remains uncertain. Most evidence indicates that individual fiber hypertrophy is the primary mechanism responsible for whole-muscle hypertrophy in humans. However, selected studies have suggested that hyperplasia may be possible in humans. In certain studies, muscle hypertrophy was observed without individual fiber hypertrophy when compared to untrained control subjects, implying a greater number of muscle fibers in the trained muscles. Yet, other studies have shown individual fiber hypertrophy in highly trained athletes compared to untrained controls.
Evidence of Hyperplasia in Humans: One longitudinal study demonstrated the possibility of hyperplasia in men with previous recreational resistance training experience. After 12 weeks of intensified resistance training, the number of muscle fibers in the biceps brachii of some subjects appeared to increase significantly, suggesting that hyperplasia can occur in humans, possibly under specific training conditions.
Satellite Cells: The formation of new muscle cells during hyperplasia is postulated to involve the division and splitting of individual muscle fibers into two daughter cells, each developing into a functional muscle fiber. Satellite cells, which are myogenic stem cells involved in skeletal muscle regeneration, likely play a role in generating new muscle fibers. These cells are typically activated by muscle stretching and injury, and muscle injury from intense training, especially eccentric-action training, can trigger satellite cell activation, proliferation, migration to damaged areas, and fusion with existing myofibers or other satellite cells to produce new muscle fibers.
Integration of Neural Activation and Fiber Hypertrophy:
Research into resistance training adaptations reveals that the early increases in voluntary strength, or maximal force production, are primarily associated with neural adaptations that enhance voluntary muscle activation. This integration of neural activation and fiber hypertrophy is crucial for understanding the mechanisms behind strength gains in resistance training.
Evidence from a Study:
A study involving both men and women who underwent an eight-week, high-intensity resistance training program (twice per week) provides valuable insights into this integration. Throughout the training period, muscle biopsies were performed at regular intervals. The results showed a substantial increase in strength, as measured by the 1-repetition maximum (1RM), with the most significant gains occurring after the second week. However, the muscle biopsies revealed only a small and statistically insignificant increase in muscle fiber cross-sectional area by the end of the eight-week training period. Therefore, the primary driver of the observed strength gains during this period was increased neural activation rather than muscle hypertrophy.
Temporal Relationship Between Neural and Hypertrophic Contributions:
Long-term increases in strength typically result from the hypertrophy of the trained muscle. Building muscle protein through a reduction in protein degradation, an increase in protein synthesis, or both, takes time. As a result, early gains in strength are predominantly attributed to changes in the neural activation pattern of muscle fibers. Most research findings suggest that neural factors play a prominent role in strength gains during the initial 8 to 10 weeks of resistance training. During these early weeks, hypertrophy contributes minimally but progressively becomes a more substantial factor, eventually becoming the primary contributor to strength gains after approximately 10 weeks of training.
Variability in Findings:
While the described pattern of strength development aligns with most research, it’s essential to note that not all studies fully concur with this pattern. Some studies have reported variations in the contributions of neural activation and hypertrophy to strength gains. For instance, a six-month study involving strength-trained athletes found that neural activation explained most of the strength gains during the most intensive training months, with hypertrophy not playing a major role in those specific conditions. This illustrates that the interplay between neural and hypertrophic adaptations can vary depending on the study design, duration, and the specific characteristics of the participants.
Muscle Atrophy and Decreased Strength With Inactivity:
When a normally active or highly trained person reduces their level of activity or ceases training altogether, major changes occur in both muscle structure and function. This is illustrated by the results of two types of studies: studies in which entire limbs have been immobilized and studies in which highly trained people stop training, a phenomenon referred to as detraining.
Immobilization: When a trained muscle suddenly becomes inactive through immobilization, significant changes are initiated within that muscle in a matter of hours. During the first 6 hours of immobilization, the rate of protein synthesis starts to decrease. This decrease is believed to initiate muscular atrophy, which is the wasting away or decrease in the size of muscle tissue. Atrophy occurs due to the lack of muscle use and the consequent loss of muscle protein that accompanies inactivity. Strength declines are most dramatic during the first week of immobilization, averaging 3% to 4% per day. This decrease in strength is associated with both muscle atrophy and decreased neuromuscular activity in the immobilized muscle.
Studies have indicated that immobilization affects both type I and type II muscle fibers. Researchers have observed disintegrated myofibrils, streaming Z-disks (discontinuity of Z-disks and fusion of the myofibrils), and mitochondrial damage as muscle atrophies. During this process, the cross-sectional fiber area decreases. Notably, the effect appears to be greater in type I fibers, leading to a decrease in the percentage of type I fibers and an increase in the relative percentage of type II fibers.
Fortunately, muscles can often recover from immobilization when activity is resumed. However, the recovery period is significantly longer than the immobilization period.
Fiber Type Alterations: Can muscle fibers change from one type to another through resistance training? Early research concluded that neither speed (anaerobic) nor endurance (aerobic) training could alter the basic fiber type, specifically from type I to type II or vice versa. However, these early studies did suggest that fibers could acquire certain characteristics of the opposite fiber type if the training stimulus was of the opposite kind. For instance, type II fibers might become more oxidative with aerobic training.
Further research using animal models has shown that fiber type conversion is indeed possible under specific conditions, such as cross-innervation. This experimental technique involves innervating a type II motor unit with a type I motor neuron or vice versa. Additionally, chronic, low-frequency nerve stimulation can transform type II motor units into type I motor units within a matter of weeks. In rodents, muscle fiber types have changed in response to 15 weeks of high-intensity treadmill training, leading to an increase in type I and type IIa fibers and a decrease in type IIx fibers. This transition of fibers has been confirmed using various histochemical techniques.
In human studies, evidence of fiber type transformation has been found in response to heavy resistance training. Women participating in a 20-week heavy resistance training program for the lower extremity experienced substantial increases in static strength and cross-sectional area across all fiber types. Notably, the mean percentage of type IIx fibers decreased significantly, while the mean percentage of type IIa fibers increased. This transition from type IIx to type IIa fibers with resistance training has been consistently reported in subsequent studies. Additionally, research has shown that a combination of high-intensity resistance training and short-interval speed work can lead to the conversion of type I to type IIa fibers.
when individuals become inactive or cease training, significant changes occur in muscle structure and function, including muscle atrophy and decreased strength. While early research suggested limited fiber type conversion, more recent studies have provided evidence of fiber type transformations in response to specific training protocols.
Muscle Soreness and Cramps:
Muscle soreness is a common experience, often resulting from exhaustive or very high-intensity exercise. It tends to be particularly pronounced when individuals perform a specific exercise for the first time. Muscle soreness can occur at various times, with a period of mild soreness during and immediately after exercise, followed by more intense soreness felt a day or two later.
Acute Muscle Soreness: The pain felt during and immediately after exercise can be attributed to several factors. One significant factor is the accumulation of metabolic byproducts of exercise, such as H+ ions. Additionally, tissue edema, which was mentioned earlier, plays a role in acute muscle soreness. Edema occurs due to fluid shifting from the blood plasma into the muscle tissues, leading to acute muscle swelling. Typically, the pain and soreness associated with acute muscle soreness subside within a few minutes to several hours after exercise. Hence, this type of soreness is often referred to as acute muscle soreness.
Delayed-Onset Muscle Soreness (DOMS): Muscle soreness that occurs a day or two after a strenuous exercise session is known as delayed-onset muscle soreness (DOMS). Although not fully understood, researchers have been working to provide a better understanding of this phenomenon. DOMS can range from mild muscle stiffness to severe, debilitating pain that limits movement.
Most contemporary theories recognize eccentric muscle actions as the primary trigger for DOMS. This has been clearly demonstrated in various studies that investigated the relationship between muscle soreness and eccentric, concentric, and static muscle actions. Individuals who exclusively train with eccentric actions often experience severe muscle soreness, whereas those using static and concentric actions tend to experience less soreness. For instance, studies have involved subjects running on a treadmill for 45 minutes on two different days, one day on a level grade and the other day on a 10% downhill grade. The downhill running, which required extensive eccentric muscle actions, resulted in considerable soreness within 24 to 48 hours, even though blood lactate concentrations (previously thought to cause muscle soreness) were much higher during level running.
The following section explores some of the proposed explanations for exercise-induced DOMS.
Structural Damage:
Intense exercise can lead to increased concentrations of specific muscle enzymes in the blood, suggesting potential structural damage to muscle membranes. These enzyme concentrations can increase from 2 to 10 times following strenuous training sessions. Recent research indicates that these changes might signify varying degrees of muscle tissue breakdown. Muscle tissue from the leg muscles of marathon runners, who engage in both training and competition, has shown notable damage to muscle fibers. The timing of these muscle changes correlates with the degree of muscle soreness experienced by the runners.
In the electron micrograph muscle fiber damage resulting from marathon running is depicted. In this case, the cell membrane appears to be completely ruptured, allowing the cell’s contents to float freely among other normal fibers. Fortunately, not all muscle cell damage is as severe.
While the effects of muscle damage on performance are not fully understood, it is generally accepted that such damage contributes, at least in part, to the localized muscle pain, tenderness, and swelling associated with delayed-onset muscle soreness (DOMS). However, it’s worth noting that blood enzyme concentrations can increase, and muscle fibers may be damaged even during daily exercise that does not result in muscle soreness. Additionally, muscle damage appears to be a contributing factor to muscle hypertrophy.
Inflammatory Reaction:
Exercise-induced muscle soreness has been linked to an inflammatory response within the muscle. White blood cells play a role in defending the body against foreign materials and conditions that threaten tissue function. Activities that induce muscle soreness tend to lead to an increase in the white blood cell count.
Early studies attempted to block the inflammatory response using drugs but were unsuccessful in reducing muscle soreness or inflammation. These results initially did not support a connection between simple inflammatory mediators and DOMS. However, more recent research has begun to establish a link between muscle soreness and inflammation.
It is now understood that substances released from injured muscle can act as attractants, initiating the inflammatory process. Mononucleated cells within the muscle become activated due to injury, providing chemical signals to circulating inflammatory cells. Neutrophils, a type of white blood cell, invade the injured site and release cytokines, which attract and activate additional inflammatory cells. Neutrophils may also release oxygen free radicals, which can damage cell membranes. The invasion of these inflammatory cells is associated with pain, likely caused by the release of substances from inflammatory cells stimulating pain-sensitive nerve endings.
Macrophages, another type of immune system cell, subsequently invade the damaged muscle fibers and remove debris through phagocytosis. Later, a second phase of macrophage invasion occurs, which is linked to muscle regeneration.
Sequence of Events in DOMS:
Delayed-onset muscle soreness (DOMS) is a complex phenomenon, and researchers have proposed a sequence of events to explain its mechanism. While a single theory cannot fully account for DOMS, the following sequence outlines some key processes involved:
- High Tension and Structural Damage: Intense muscular tension, particularly during eccentric (lengthening) muscle actions, leads to structural damage within the muscle and its cell membrane. This process may also involve excessive strain on the connective tissue.
- Disturbed Calcium Homeostasis: Damage to the cell membrane disrupts calcium homeostasis within the injured muscle fiber. This disruption inhibits cellular respiration and results in elevated calcium concentrations within the fiber. High calcium levels activate enzymes that degrade the Z-lines, which are structural components of muscle fibers.
- Inflammatory Response: Within a few hours after the initial damage, there is a significant increase in circulating neutrophils, a type of white blood cell. Neutrophils are part of the inflammatory response and may contribute to the release of inflammatory mediators and cell signaling in the injured muscle.
- Accumulation of Substances and Nerve Stimulation: The activity of macrophages, another type of immune cell, and the release of intracellular contents, including histamine, kinins, and potassium ions (K+), accumulate outside the muscle cells. These substances can stimulate free nerve endings within the muscle tissue, contributing to the sensation of pain and discomfort. This process may be more pronounced in eccentric exercise, where large forces are distributed over relatively small cross-sectional areas of the muscle.
Recent comprehensive reviews have shed more light on the causes of muscle soreness. It is now understood that muscle soreness results from injury or damage to the muscle, primarily affecting the muscle fibers and possibly the cell membrane. This damage triggers a series of events that involve the release of intracellular proteins and an increase in muscle protein turnover. Several factors, including calcium ions, lysosomes, connective tissue, free radicals, energy sources, inflammatory reactions, and intracellular and myofibrillar proteins, play roles in the damage and repair process. However, the exact cause of skeletal muscle damage and the mechanisms of repair are not fully understood. Some evidence suggests that this process is a crucial step in muscle hypertrophy.
In addition to muscle injury, edema, or the accumulation of fluids within the muscle compartment, can also lead to DOMS. Edema is likely a result of muscle injury but can potentially occur independently of muscle damage. The buildup of interstitial or intracellular fluid increases tissue fluid pressure within the muscle compartment, activating pain receptors within the muscle and contributing to the sensation of soreness.
Delayed-Onset Muscle Soreness (DOMS) and Performance:
DOMS not only causes discomfort but also leads to a reduction in the force-generating capacity of the affected muscles. When individuals with DOMS are asked to exert maximal force, such as in a 1-repetition maximum (1RM) strength test, their affected muscles are unable to generate as much force as usual. This reduction in strength is temporary and gradually returns to normal over the course of days or weeks. The loss in strength during DOMS can be attributed to three key factors:
- Physical Muscle Disruption: The physical damage to the muscle, plays a role in the loss of strength.
- Failure in Excitation-Contraction Coupling: Failure within the excitation-contraction coupling process, particularly during the first five days of DOMS, is a crucial factor contributing to reduced strength.
- Loss of Contractile Protein: There is also a loss of contractile protein in the affected muscle during DOMS.
Excitation-contraction coupling refers to the process by which electrical signals (excitation) lead to muscle contraction (contraction). Failure in this process impairs the ability of muscle fibers to respond to neural signals, resulting in reduced force production.
Furthermore, muscle glycogen resynthesis is impaired when a muscle is damaged. Although glycogen resynthesis is initially normal in the hours following exercise, it slows or stops as the muscle undergoes repair, limiting the fuel-storage capacity of the injured muscle.
To illustrate the time sequence of various factors associated with intense eccentric exercise (which often leads to DOMS), including pain, edema, plasma creatine kinase (a marker of muscle fiber damage), glycogen depletion, ultrastructural muscle damage, and muscular weakness.
Reducing the Negative Effects of DOMS: Reducing the negative effects of DOMS is important for optimizing training outcomes. Several strategies can be employed to mitigate the impact of DOMS:
- Gradual Progression: Initiate training at a low intensity and progress slowly during the first few weeks. This gradual approach can help reduce the severity of DOMS.
- High-Intensity Start: Alternatively, some athletes may choose to begin their training program with a high-intensity, exhaustive bout of exercise. While this may result in significant soreness initially, subsequent training sessions are likely to cause less soreness. This approach is based on the idea that DOMS is necessary to stimulate muscle hypertrophy.
- Conditioning: Maintain good physical conditioning to reduce the likelihood of muscle fatigue and excessive strain on muscles.
- Stretching: Regularly stretch muscle groups prone to DOMS to improve flexibility and reduce the risk of soreness.
- Hydration and Nutrition: Maintain fluid and electrolyte balance, as well as carbohydrate stores, to support muscle function and recovery.
- Adjust Exercise Intensity: Reduce exercise intensity and duration if necessary to prevent excessive muscle damage and soreness.