Basic Mechanical Principles
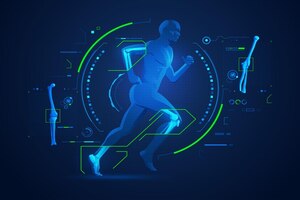
This article provides an essential foundation in basic mechanical concepts that significantly impact athletic performance. It focuses on fundamental principles and introduces readers to the critical influence of Earth’s gravitational pull on various sports activities. Understanding these mechanical concepts is essential for coaches, athletes, and sport enthusiasts.
Gravitational Force: The article begins by discussing the role of gravity in sports. While gravity can assist some athletes, it poses a significant challenge in sports like jumping and throwing. Readers learn about the characteristics of gravity, including its impact on an athlete’s body mass, body weight, and inertia.
Body Mass vs. Body Weight: The distinction between body mass and body weight is clarified. Mass refers to the quantity of matter an object possesses and is typically measured in pounds (lb) or kilograms (kg). Weight, on the other hand, incorporates the gravitational force acting on an object. For athletes, body mass is a crucial parameter to monitor. Athletes understand that having the right body mass is essential for optimal performance, as having too much or too little mass can significantly affect their abilities. The article emphasizes that while mass is a fundamental term, weight is the force generated by this mass, calculated by multiplying mass by the acceleration due to gravity (typically 10 m/s² on Earth). Athletes often monitor their body mass as part of their training and performance evaluation.
Effects of Body Mass on Athletes: The article also discusses the significance of an athlete’s body mass, especially in sports like American football where linemen are often described as massive. Body mass consists of muscle, bones, fat, tissue, fluids, and other substances, and athletes must carefully manage their body mass to optimize their performance. The article emphasizes that body mass plays a crucial role in an athlete’s ability to excel in their chosen event.
Weight and Gravity: In mechanical terms, an athlete’s weight is described as the gravitational force exerted by the Earth on the athlete’s body. The scale’s reading represents the extent of this gravitational pull. Athletes with more body mass compress the springs of a scale more, leading to a higher reading on the scale. In essence, an athlete’s weight is a measure of the gravitational force that the Earth exerts on them.
This article lays the groundwork for understanding the significance of body mass and weight in sports. Coaches and athletes are introduced to these fundamental mechanical principles, which are vital for comprehending sports mechanics and enhancing athletic performance. The knowledge of how gravity affects body mass and weight is crucial for coaches and athletes as they strive to optimize performance in different sports activities.
This part delves into the concept of inertia, which plays a crucial role in understanding sports mechanics. Inertia goes beyond its common usage, which often relates to people being slow to take action. In mechanical terms, inertia refers to an object’s, or an athlete’s, resistance to changes in motion. It’s the “desire” of an object to persist in its current state of motion or rest, resisting any alterations.
Inertia and Mass: Mass is a key determinant of inertia. The greater an object’s mass, the more inertia it possesses. The article provides a practical example: comparing the ease of getting a men’s shot put (16 lb or 7.3 kg) moving compared to a tennis ball (2 oz or 56 g). Naturally, it’s harder to initiate motion in the shot put due to its greater mass. Moreover, objects in motion prefer to continue in a straight line unless external forces act upon them. For instance, a baseball thrown by an outfielder initially moves along its release trajectory, but air resistance and gravity alter its path.
Impact of Athlete Mass and Inertia: Athletes with larger body masses have more inertia, and this resistance to change affects their performance. Giant athletes (e.g., sumo wrestlers or American football linemen) must exert significant force to get their mass moving and require immense effort to stop or change direction. In contrast, athletes with less body mass experience less inertia and can initiate and control their movements more easily.
Inertia in Sports: In sports like squash or badminton, massive athletes may encounter limitations. Sudden and varied movement changes can be challenging for large, heavy athletes if they lack the ability to move quickly and control their mass. These sports often favor lean and lightweight players who can use their opponent’s inertia against them. Smaller players can enjoy making their massive opponents crash into walls.
Inertia in Flight: When athletes are in flight, as in bungee jumping, the effects of inertia are evident. Two athletes of different masses jumping from the same height accelerate toward the Earth at roughly the same rate. The more massive athlete experiences greater gravitational attraction but also greater inertia, balancing out the acceleration effects. Air resistance has a minimal role in this scenario.
Inertia as an Enemy and a Friend: Inertia can act as an obstacle when an athlete initially attempts to move. Athletes overcome inertia with strong, powerful muscles that can generate the necessary force to initiate motion. Once in motion, inertia becomes an ally as it motivates the athlete to persist in their current state of motion.
Rotary Inertia: The concept of inertia applies not only to linear motion but also to rotary motion. Rotary inertia, also known as rotary resistance or moment of inertia, involves more than just the mass of an object. It considers how mass is distributed concerning the axis around which the object is spinning.
Understanding inertia is essential in sports mechanics because it influences how athletes move and interact with their surroundings. Inertia plays a role in linear and rotary situations, affecting an athlete’s ability to initiate, maintain, or change motion. Knowledge of inertia is fundamental for coaches and athletes, helping them optimize performance and technique while considering the effects of mass and inertia in different sports and scenarios.
This part explores different types of motion in the context of sports mechanics: linear, angular, and general motion. Understanding these types of motion is essential for analyzing and optimizing athletic performance.
Linear Motion: Linear motion refers to movement that occurs in a straight line. It can also be called translation. However, in sports, true linear motion is rare because different parts of an athlete’s body often move at varying speeds and not always in the same direction. For example, in a 100-meter sprint, athletes aim to travel the shortest distance in a straight line, but their movements are primarily produced by the rotation of limbs around joints, and their center of gravity rises and falls during each stride.
Angular Motion: Angular motion plays a dominant role in sports because most of an athlete’s movements involve the rotation or swinging action of the limbs around joints. Terms like rotating, spinning, swinging, circling, turning, rolling, pirouetting, somersaulting, and twisting are used to describe angular motion. Athletes may turn through specific angles, such as quarter turns (90 degrees), half turns (180 degrees), and full turns (multiples of 360 degrees). In sports like gymnastics, skateboarding, basketball, diving, figure skating, and ballet, athletes perform various angular movements.
Axes of Rotation: Angular motion occurs around an axis, similar to an axle or hinge. An athlete’s body has many joints that act as axes for rotation. The upper arm rotates at the shoulder joint, the lower arm at the elbow joint, and the hand at the wrist. Similarly, the hip joint, knee joint, and ankle joint serve as axes for the leg’s rotational motion. Walking and running depend on the rotary motion of each limb segment as they rotate around these joints.
General Motion: All human motion can be described as general motion, which is a combination of linear and angular motion. Even sport skills that require athletes to maintain specific positions involve some level of both linear and angular motion. For instance, a gymnast balancing on a beam or a ski jumper crouching during takeoff exhibits a mix of linear and angular motion. In a wheelchair race, athletes demonstrate a visible combination of angular motion in the repetitive arm movements and linear motion as the wheels carry the athlete and chair along the track.
Understanding the distinctions between linear, angular, and general motion is vital for coaches and athletes. It provides insights into how the body’s various movements can be optimized for different sports and activities. Recognizing these principles helps in the analysis of athletic performance and the improvement of techniques and strategies.
This article delves into the concepts of speed, velocity, and acceleration and how they relate to sports mechanics. These are fundamental aspects of motion analysis in sports.
Speed and Velocity: Speed and velocity are terms often used interchangeably but have distinct differences. Speed is a scalar measure that indicates how fast an object is traveling concerning time. It is calculated by dividing the distance traveled by the time taken. However, speed does not account for the direction of travel. On the other hand, velocity is a vector measure representing the change in position divided by time. Velocity accounts for both speed and direction. For instance, if an elite sprinter runs 100 meters in 10 seconds in a straight line, both the speed and velocity are the same because the direction remains constant. However, when the direction matters, such as in kicking a ball, we use velocity to describe the object’s speed and direction.
It’s crucial to understand the difference between speed and velocity in sports to describe motion accurately. While speed provides a measure of how fast an athlete moves, velocity adds the dimension of direction, which is vital in various sports scenarios.
Acceleration and Deceleration: Acceleration is the rate at which an athlete’s velocity or speed changes. Athletes do not maintain a constant speed throughout their performance; instead, their speed can increase, decrease, or remain constant during different phases of an event. Acceleration, or its opposite, deceleration, varies among athletes. The rate at which an athlete accelerates or decelerates can be a significant factor in their performance. Some athletes exhibit high acceleration at the start of a race, which then decreases, while others may continue to accelerate even into the latter part of the event.
Acceleration is measured in units of distance per time squared (e.g., feet per second squared or meters per second squared). Athletes’ velocity increases or decreases in response to acceleration or deceleration. Uniform acceleration and uniform deceleration are concepts wherein the athlete’s speed increases or decreases at a constant rate. These are relatively rare in sports as many factors contribute to nonuniform changes in speed, such as oppositional forces and air resistance. However, they are often observed in activities like high jump, long jump, diving, trampoline, and gymnastics, where air resistance is minimal and gravity uniformly affects the athlete’s speed.
In such cases, when athletes rise in the air, they decelerate uniformly, and their speed decreases at a consistent rate. When they descend, gravity accelerates them at a uniform rate. These uniform changes in speed can be measured using acceleration units.
Understanding speed, velocity, and acceleration is vital for coaches and athletes to analyze and improve performance. It helps in deciphering an athlete’s speed fluctuations, identifying areas for improvement, and developing strategies to maximize performance.
How to measure speed, velocity, and acceleration in sports, both manually and with the help of technology.
Manual Measurement of Linear Velocity:
- To measure velocity manually, you need a tape measure and a stopwatch. This method works well for linear movements, such as a 100m sprint.
- A predefined distance, like every 10 meters, is established. You measure the time it takes for the athlete to traverse this distance.
- In the spreadsheet, the first column contains the predefined distance intervals, like every 10 meters. The second column records the time it takes for the athlete to pass through this distance.
- You can calculate additional variables using the information from the first two columns. The third column represents the change in time to cover that 10m interval, called the time-split.
- The fourth column calculates velocity, which is the distance (10m) divided by the time-split needed to cover that interval.
- The fifth column shows the difference in velocity between each interval, and the sixth column calculates acceleration, which is the change in velocity divided by the time split.
Using Technology to Measure Linear Velocity:
- Various technologies can automate the process of measuring linear velocity. One such technology is timing gates, which employ a laser or infrared beam and a reflective marker.
- As the athlete crosses the beam, the device triggers an internal stopwatch to record the time. You can then measure the distance between the reflective markers to calculate the runner’s speed or velocity.
- Timing gates are especially useful in team sports, where automated speed and velocity calculations save time and reduce the likelihood of errors.
- Modern radar equipment, often used in sport research, provides precise data about the speed and distance of athletes or objects.
- Radar equipment can determine the speed of a baseball as it leaves the pitcher’s hand or as it leaves the bat upon being hit. It can also calculate the distance over which a sprinter accelerated, maintained a particular speed, or decelerated during a race.
These methods and technologies enable coaches, athletes, and researchers to obtain accurate data on speed, velocity, and acceleration, helping them better understand and improve athletic performance.
Gravity as an Invisible Force:
- Gravity is an invisible force that is omnipresent on Earth, and it is sometimes challenging to grasp due to its intangibility.
- There are slight variations in gravitational forces at different places on Earth, which can have effects on sports performances.
Impact of Location on Gravity:
- The location where the Olympic Games are held illustrates how gravity can differ. For instance, the 1968 Olympics in Mexico City, situated at a higher altitude and closer to the equator, experienced slightly less gravitational pull compared to the 1952 Olympics in Helsinki or the 1980 Games in Moscow, both at northern latitudes and closer to sea level.
- Peter Brancazio, a physics professor at Brooklyn College, considered gravity independently of air resistance. He calculated that a 70-foot shot put in Oslo, Norway (latitude 60 degrees N), would travel 1 inch (25 mm) farther in Montreal, Canada (45 degrees N); 2 inches (50 mm) farther in Cairo, Egypt (30 degrees N); and 3 inches (75 mm) farther in Caracas, Venezuela (10 degrees N). Similarly, a 300-foot (91.4 m) javelin throw in Moscow (56 degrees N) would travel 301 feet (91.7 m) in Lima, Peru (12 degrees S).
Thin Air at High Altitudes:
- At high altitudes, including Mexico City, there’s what’s known as “thin air.” While the composition of air at high altitudes is the same as at sea level (21 percent oxygen, 78 percent nitrogen, and 1 percent other gases), there’s less of each gas per unit of volume due to the lower atmospheric pressure at higher altitudes.
- In sports competitions at high altitudes, athletes must breathe more vigorously and frequently to obtain sufficient oxygen. This can pose challenges for endurance events but can be advantageous for short sprints because sprinters primarily rely on stored energy.
- The 1968 Olympic Games in Mexico City demonstrated the impact of thin air, with both its challenges for endurance athletes and benefits for sprinters.
- Bob Beamon, who set a long jump world record in Mexico City, benefited from the reduction in gravity, lower air resistance due to less dense air, and the fact that his long jump approach was more of a short sprint than a distance run.
- It’s noted that Mike Powell later broke Bob Beamon’s long jump record at a lower altitude in Tokyo. However, this performance may have produced an even greater distance if it had taken place in Mexico City due to differences in atmospheric conditions.
- Tokyo’s track surface, which contributed to the record, was subsequently banned for enhancing athletes’ performances, including long jumpers.
In summary, gravity’s strength and the thinness of air at high altitudes can impact athletes’ performances in various ways, making location an important consideration in sports events and world records.
Acceleration Due to Gravity:
- When a pole-vaulter drops from above the bar, the force of gravity accelerates the athlete toward the landing pit. If the vaulter clears the bar at a greater height, the increased distance provides more time for gravity to accelerate the athlete on the way down. Thus, a pole-vaulter descending from a height of 20 feet hits the pit at a higher velocity compared to a vaulter clearing the bar at 15 feet.
- A similar gravitational acceleration experience is encountered by tower divers as they fall toward the water. Earth’s gravitational pull continuously accelerates divers during their descent.
Uniform Acceleration Due to Gravity:
- An athlete steps off and drops from a height of 256 feet.
- After 1 second of free fall, the athlete reaches a velocity of 32 feet per second (9.8 meters per second), equivalent to approximately 21.8 miles per hour (35 kilometers per hour).
- At 2 seconds, the velocity has doubled to 64 feet per second (19.6 meters per second) or about 43.6 miles per hour (70 kilometers per hour).
- By 3 seconds, the velocity has tripled to 96 feet per second (29.4 meters per second) or around 65.4 miles per hour (105 kilometers per hour).
- Finally, after 4 seconds, the athlete’s velocity has quadrupled to an incredible 128 feet per second (39.2 meters per second) or roughly 87.2 miles per hour (140 kilometers per hour).
- Gravity uniformly accelerates the falling athlete at a rate of 32 feet per second (9.8 meters per second) for every second of descent, or 32 feet per second squared (9.8 meters per second squared).
Impact of Acceleration on Distance:
- The continuous increase in velocity due to uniform gravitational acceleration leads to an athlete covering increasingly large distances with each passing second of free fall.
- This remarkable acceleration emphasizes the inherent risks in sports such as tower diving.
Air Resistance and Terminal Velocity:
- Tower divers reach high speeds and enter the water surface at approximately 38 miles per hour (61 kilometers per hour) after a short descent of 10 meters.
- In the case of parachutists, they don’t experience continuous acceleration toward the Earth because air resistance becomes significant. As a result, parachutists in free fall reach a constant, or terminal, velocity of approximately 125 miles per hour (201 kilometers per hour).
- Variations in terminal velocity can occur based on body positions used by the parachutist during free fall. For instance, a stomach-down, spread-eagle position results in a slower terminal velocity compared to a headfirst, legs-together position.
- The type of parachute also affects the speed at which the parachutist descends. Modern wing-shaped, ram-air parachutes offer greater maneuverability and softer landings compared to older half-sphere parachutes.
In summary, the force of gravity causes uniform acceleration during free fall, leading to increased velocity and distance covered over time. However, air resistance plays a significant role in determining terminal velocity in scenarios like parachute jumps.
An Athlete’s Center of Gravity:
- Gravity, as discussed previously, exerts a force on everything with mass, pulling it toward the Earth’s center. This force acts consistently on all parts of an object.
- Just as we can measure an athlete’s overall velocity when running, we can also measure the velocities of individual components of their body, such as arms or legs.
- By collectively summing up all the components of an object, we can find a balance point, known as the object’s center of gravity. This is a theoretical point used for calculations and understanding an object’s movement.
- Consider a wooden baseball bat. If each particle of wood in the bat has the same mass, the gravitational force from the Earth affects each particle equally. When these forces are combined, the location where this force is concentrated is considered the bat’s center of gravity. In a baseball bat, this center of gravity is often referred to as the “sweet spot.”
- Another example is a wooden ruler used for measuring short distances. You can balance it on your fingertip at a point halfway in from the ends and halfway in from the sides, where there are equal amounts of mass lengthwise and widthwise from the center of gravity.
- If you attach a small piece of lead to one end of the ruler, you must move your supporting finger closer to the lead to maintain balance. The lead, with its concentrated mass, balances a longer section of the ruler on the other side.
- The same principle applies to balancing a metal hammer with a wooden handle horizontally on your finger. The balance point will be close to the hammer’s head.
Athlete’s Body and Center of Gravity:
- An athlete’s body is not uniform in composition. It consists of different substances, including bone, muscle, fat, and tissue, each with different densities and shapes.
- Density refers to the mass contained within a specific volume. Bones and muscles have higher density and more mass concentrated in their space compared to an equal volume of body fat.
- Because an athlete’s body is made up of materials with varying densities, the center of gravity is not equidistant from the head and feet.
- However, the athlete’s center of gravity naturally adjusts itself so that there is as much mass directly above it as there is below it, and as much mass to the left as there is to the right.
In summary, an athlete’s center of gravity is the point where the combined gravitational forces act. It adjusts based on the distribution of mass within the athlete’s body, ensuring that there is equilibrium both vertically and horizontally, despite variations in density and shape. Understanding an athlete’s center of gravity is essential for analyzing their movements in various sports.
Shifts in the Center of Gravity:
This section discusses how athletes can shift their center of gravity to optimize their performance. The center of gravity is a critical concept in understanding how athletes maintain balance and execute movements in various sports.
- In sports, athletes often shift their center of gravity from one position to another to achieve optimal performance. This involves strategically positioning their center of gravity to ensure stability while applying force or executing movements.
- During a golf drive, for example, athletes shift their body weight from the rear foot to the forward foot, effectively repositioning their center of gravity to improve stability when striking the ball.
- Athletes, whether on land, in the air, or in the water, employ these principles to reposition their center of gravity.
Factors Affecting the Center of Gravity:
- The natural position of the center of gravity for males, when standing upright with arms by their sides and palms facing forward, is around the belly button or navel. However, this position can shift up or down depending on the distribution of mass in the upper body (e.g., chest and shoulders) and lower body (e.g., thighs).
- There are anatomical differences between males and females that influence the position of their centers of gravity. Males tend to have more mass in the shoulders and less in the hips, while females exhibit the reverse pattern.
- Factors such as body composition, including the distribution of muscle and fat, can cause variations in an athlete’s center of gravity. For instance, an athlete with heavily muscled legs and a lighter upper body will have a lower center of gravity.
- Athletes can also modify their center of gravity through training. For instance, bodybuilders who focus on developing their upper body may shift their center of gravity upwards.
- Athletes can shift their center of gravity through limb movement. When an athlete moves a leg forward to take a step, the center of gravity shifts in the same direction. Moving both a leg and an arm further shifts the center of gravity. The extent of the shift depends on the amount of mass moved and the distance over which it is moved.
Center of Gravity Outside the Body:
- In certain situations, athletes can shift their center of gravity outside their bodies. For instance, when a gymnast performs a high back arch or a back walkover, they shift their center of gravity outside their body as they flex at the waist. This can also be observed in divers performing various positions.
- The degree of shift in the center of gravity depends on the extent of the movement and the shift in mass distribution in the legs, upper body, and arms.
Sport-Specific Center of Gravity Positioning:
- Athletes position their center of gravity based on the specific demands of their sport. For example, wrestlers and judo practitioners constantly reposition their center of gravity to gain stability in response to their opponents’ actions.
- In sprint races, athletes shift their center of gravity in the direction they intend to sprint, ensuring a swift response when the race begins.
- In gymnastics, balance beam athletes work to maintain their center of gravity directly above the beam.
- Athletes in track and field throwing events position their center of gravity to apply the maximum force to the implement over the greatest time.
In summary, an athlete’s ability to control and shift their center of gravity is a fundamental aspect of performance in various sports. Precise positioning of the center of gravity is crucial for maintaining balance and optimizing movements specific to each sport.
How Gravity Affects Flight:
In sports involving short periods of flight, such as the high jump, long jump, gymnastics, figure skating, trampoline, and diving, the athlete’s flight path is established at takeoff. This flight path is influenced by the application of forces in both vertical and horizontal directions. The resultant of these forces determines the athlete’s takeoff trajectory.
Flight Path and Gravity:
- While in flight, the athlete is subjected to the gravitational pull of the Earth, much like on the ground. This gravitational force acts on the athlete’s center of gravity and imparts a downward pull, resulting in the characteristic parabolic (curved) flight path.
- Once an athlete is airborne, they have no means to alter the flight path that was initially set during takeoff. Any errors in the takeoff phase can have significant consequences for the athlete’s trajectory.
Vertical and Horizontal Thrust:
- In flight, the athlete experiences both vertical and horizontal thrust. The combination of these forces defines the takeoff trajectory. For example, in diving, if a diver thrusts predominantly in the vertical direction, it can lead to a return to the takeoff board or a trajectory too close to the horizontal, impeding the execution of twists and somersaults.
Flight Control:
- In certain sports, a high degree of flexibility can allow an athlete’s center of gravity to pass under an obstacle, such as a high jump bar, while their body maneuvers over the top. This technique is advantageous as it reduces the height the athlete’s center of gravity needs to reach to clear a particular height.
Comparison in Techniques:
- The text compares techniques used by athletes in different sports to illustrate the significance of a well-controlled flight path. For example, a high jumper using a “flop” technique can clear a certain height with less elevation of their center of gravity compared to a “squat jump” technique.
- Trampolinists aim to ascend vertically above the trampoline’s midpoint and then descend in a controlled manner, minimizing horizontal travel along the trampoline bed. In contrast, divers, especially springboard divers, must train to avoid contact with the diving board during their flight path.
Examples of Flight Path:
- The narrative provides examples of athletes such as high jumpers and divers who must manage their flight paths carefully to achieve success. In the case of divers, even exceptional athletes like Greg Louganis have faced penalties for inadequate clearance from the diving board.
In summary, how gravity affects flight is a critical consideration in sports with short periods of aerial activity. Athletes must understand the forces acting on their center of gravity and manipulate their takeoff technique to achieve the desired flight path. Once in the air, they have no means to alter their trajectory, making precise takeoff crucial to their performance.
May the Force Be With You
This text delves into the fundamental concept of force in the realm of athletics and explores how it influences an athlete’s performance. It introduces the invisible yet influential nature of force and its role in driving motion and reactions in sports. The text unfolds in a logical sequence:
Understanding the Nature of Force:
- It begins by emphasizing the necessity of force for an object to either move or remain stationary. The presence of force is presented as a fundamental requirement.
- The concept of force is defined as a push or a pull that has the capacity to change an object’s shape or its state of motion. The text highlights that while force itself is not visible, its effects can be observed and experienced.
Illustration through Examples:
- The text employs a vivid example of a weightlifter attempting to lift a barbell in a vertical direction. It explains that if the weightlifter applies sufficient force, the barbell is lifted. In the absence of enough force, there remains a “tendency” for the barbell to move due to the force exerted by the athlete, even if it doesn’t initiate motion.
- It elaborates on how combining the force of multiple athletes pulling in the same direction can result in moving an object. The direction of the applied force is highlighted as a critical factor.
- The influence of mass and gravitational force on Earth is explained. It is noted that athletes, due to their mass, pull upward on Earth while simultaneously being pulled downward by Earth’s gravity. The equal and opposite forces are crucial in understanding the interaction between athletes and the ground.
Equal and Opposite Reactions:
- The text introduces the concept of the ground reaction force, which is the force exerted by the ground in reaction to an athlete’s downward pressure. This force aligns with Isaac Newton’s third law, stating that every action has an equal and opposite reaction.
- Examples of visible actions and reactions, such as the recoil of a fired rifle and the deflection of air during a jetliner’s takeoff, are provided to illustrate the principle.
- A relatable example of a player punching a locker is presented to showcase how the locker “punches back” as a reaction to the player’s action.
Role of Friction and Traction:
- The text discusses the importance of friction and traction in sports and how athletes adjust the pressure they exert on surfaces to control these factors. Snowboarding serves as an example, with variations in pressure aiding in carving turns and adapting to terrain changes.
- It explains how the quick reduction in friction and ground reaction force can enable rapid directional movements.
- The relationship between leg flexion and extension and the resulting effects on pressure, friction, and movement is detailed, demonstrating the importance of these principles in snowboarding and skiing.
Equal and Opposite Reactions in Flight:
- The text highlights that in flight, athletes more readily observe how every action generates equal and opposite reactions. Movements of one part of the body result in visible reactions in another part.
- The application of this law of equal and opposite reactions is demonstrated in sports like diving, gymnastics, trampolining, and trapeze acts.
Force Vectors
This text explores the concept of force vectors in the context of sports and mechanics, discussing how they represent the combination of forces with known directions and magnitudes. The explanation is as follows:
Understanding Force Vectors:
- The text begins by revisiting the weightlifting scenario mentioned earlier and highlights the combination of forces applied by two lifters to lift a barbell.
- It introduces the concept of a force vector, explaining that a force vector is a quantity with direction. In the case of weightlifting, the force vector represents the amount of force applied in a specific direction, such as vertically.
Representation of Force Vectors:
- Force vectors are often depicted diagrammatically using arrows in mechanics. The head of the arrow indicates the direction of the force, and the length of the arrow represents the magnitude of the applied force.
- The text uses the weightlifting example again to illustrate that when one athlete lifts the barbell vertically and the other pulls it horizontally, the combination of their forces results in the barbell moving both upward and sideways, determined by the resultant force vector.
Calculation of Resultant Force:
- The process of combining two forces applied in different directions is visually depicted using two athletes pulling on a large resistance. Athlete A applies a force toward the north, while athlete B applies a force toward the east. The dimensions of their applied forces are provided, and a parallelogram is drawn to represent the resultant force vector.
- The text explains that careful measurement reveals the magnitude and direction of the resultant force vector, which results from the combined efforts of the two athletes.
Practical Application of Force Vectors:
- The text transitions to practical examples in sports, such as the shot-put event. It describes how athletes apply both vertical and horizontal forces to give the shot a specific trajectory, with the angle between the two components determining the desired trajectory.
- The influence of gravity and air resistance on the shot’s trajectory is highlighted. Gravity primarily opposes the vertical force component, while air resistance affects the shot’s forward motion.
Sports Scenarios Involving Force Vectors:
- The text draws parallels with other sports, such as soccer, football, and basketball, where athletes make precise passes to teammates based on vector analysis. They factor in variables like wind direction and the speed of their teammates to ensure the ball reaches the desired location.
- The internal vector forces within the human musculoskeletal system are mentioned, highlighting that the human body also generates internal forces resulting in external movements. Depending on the orientation of anatomical limbs, these internal vector forces can create both vertical and horizontal components.
In summary, this text provides an explanation of force vectors, their representation, and practical applications in sports. It illustrates how forces with known directions and magnitudes combine to create specific movement patterns, emphasizing their significance in various athletic scenarios.
Projectiles
In this section, the text explores the concept of projectiles in various sports and activities. It discusses how athletes or objects are propelled through the air, emphasizing the need for control and assessment of the flight path. The description is as follows:
Understanding Projectiles:
- The text introduces the idea that in many sports and activities, athletes and objects are projected or propelled through the air. It provides examples such as golf balls, basketballs, baseballs, javelins, as well as athletes in sports like ski jumping, trampoline, trapeze, and diving. In all these cases, manipulation, control, or assessment of the flight path is crucial.
Examples of Flight Path Control:
- The text proceeds to offer specific examples of how athletes in different sports manipulate and control flight paths. For instance, an archer adjusts the bow and string to ensure the arrow hits the bull’s-eye accurately. A high jumper aims for specific height, distance, and rotation to clear the bar successfully. Divers seek a flight path that allows them enough airtime to perform twists and somersaults while positioning for a splashless entry. Goalkeepers evaluate the velocity and flight path of balls or pucks to make successful saves, and tennis players aim to land the ball in a location that challenges their opponent’s return.
Factors Influencing Flight Path:
- The text explains that in events involving flight, various factors come into play to influence the character of the flight path.
- Athletes take off at specific angles in jumping events, which affect the trajectory of their flight.
- Baseballs are given particular trajectory angles during pitches, throws, or hits, determining their path.
- Jumpers can vary the speed of their takeoff, affecting the trajectory they follow.
- The speed at which a baseball comes off the bat or is released from the pitcher’s or fielder’s hand can also vary, impacting its flight path.
- The height from which an athlete takes off or from which a baseball is hit or thrown is another factor that influences the flight path.
In summary, this text delves into the concept of projectiles in sports and activities, emphasizing the importance of controlling and assessing the flight path. It provides examples of how athletes in different disciplines manage flight trajectories and discusses various factors that influence these paths, from launch angles to release speeds and heights.
Trajectory
In this section, the text discusses the concept of trajectory, particularly in the context of the flight of a baseball. It breaks down the factors that influence a projectile’s path, including the angle of release, speed of release, and height of release. The interaction of these factors is explored, and their impact on the flight path is explained. Here’s a comprehensive description:
Understanding Trajectory:
- The section begins by addressing how various factors interplay in understanding trajectory, specifically in the case of a baseball’s flight. It initially sets aside the effects of gravity and air resistance to simplify the analysis.
Eliminating External Forces:
- To simplify the analysis, the text starts by eliminating the influence of gravity and air resistance. It introduces the scenario of a pitcher throwing a baseball at a 35-degree angle above the horizontal. To achieve this trajectory, the pitcher applies slightly more force in the horizontal direction than in the vertical direction.
Newton’s First Law:
- The text mentions that, without gravity and air resistance, a baseball released at a 35-degree angle will continue indefinitely in its upward trajectory at the initial release speed. This behavior aligns with Newton’s first law of inertia.
Reality with Gravity and Air Resistance:
- The text then acknowledges that in reality, gravity pulls the baseball toward the Earth, while air resistance counteracts its forward motion, causing the familiar curved flight path where the ball ascends to a point and then descends back to the Earth’s surface.
Gravity’s Influence:
- It emphasizes that gravity primarily affects the vertical component of the force applied to the ball, leading to the ball’s rise and subsequent fall.
Factors Affecting Distance and Trajectory:
- The section proceeds to delve into the factors that influence the distance and trajectory of a projectile. It explains that three critical factors play a role: angle of release, speed of release, and height of release.
Angle of Release:
- It describes how the angle at which an athlete releases a ball significantly impacts the flight path. The text categorizes three main types of flight paths based on the angle of release, including straight up, height-dominated, and distance-dominated trajectories.
Speed of Release:
- The text delves into the effect of varying the speed of release. It states that a higher release speed results in a higher apex (the highest point in the flight path) for a straight-up throw, and increased speed for other angles leads to both higher release points and longer travel distances.
Height of Release:
- The height of release concerning the surface on which the projectile lands is discussed. The text mentions that different sports and activities involve different release heights, such as golfers hitting from ground level, baseball players releasing above ground level, or shot-putters releasing the shot above shoulder level. The relationship between release height and trajectory angle is explored.
Compromises in Sports:
- The text provides examples of athletes, such as long jumpers, who need to compromise between velocity at takeoff and takeoff angle. It explains that a balance between these factors is crucial in achieving the best results.
Interplay of Factors:
- The section concludes by highlighting the interrelated nature of velocity, height, and angle of release. Changes in one component lead to adjustments in the others. Furthermore, it mentions that aerodynamic and environmental factors, like wind direction and velocity, also affect the flight path of projectiles and athletes, such as those in the javelin, discus, and skiing.