Muscle Physiology
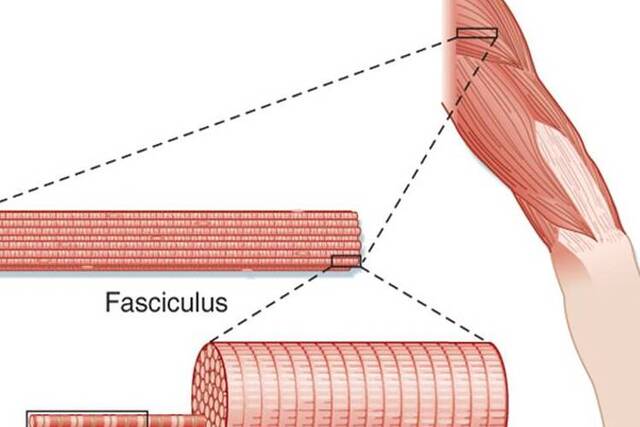
The following sections provide an in-depth exploration of the architectural organization of skeletal muscles, emphasizing both their macroscopic and microscopic structures. Additionally, we delve into the sequential chemical and mechanical events that govern muscle action and relaxation. Furthermore, we highlight distinctions in muscle fiber characteristics between sedentary individuals and elite athletes involved in various sports.
Gross Structure of Skeletal Muscle
Skeletal muscles, numbering over 660 in the human body, exhibit a complex structural organization characterized by fibrous connective tissues. . Individual fiber length can vary, ranging from a few millimeters in muscles controlling eye movement to nearly 30 cm in the robust antigravity muscles of the legs, with a width of up to 0.15 mm.
The structural organization of skeletal muscle can be described at various levels:
- Endomysium: This fine layer of connective tissue envelops each muscle fiber, providing separation from neighboring fibers.
- Perimysium: Bundles of up to 150 muscle fibers, called fasciculi, are surrounded by the perimysium.
- Epimysium: The entire muscle is ensheathed by the epimysium, a fibrous connective tissue fascia. At its ends, the epimysium tapers and blends with intramuscular tissue sheaths to create strong, dense connective tissue structures known as tendons. These tendons anchor the muscle to the periosteum, the outer covering of bones. The tendon’s tissues intertwine with collagen fibers within the bone, forming a robust connection between muscle and bone. This connection is typically unyielding, except under severe stress when it may rupture or detach from the bone.
- Muscle Origin and Insertion: Muscles have an origin, where the tendon joins a relatively stable skeletal part, typically the proximal or fixed end of the lever system or the part closest to the body’s midline. The point of attachment to the moving bone represents the insertion of the muscle.
The force generated during muscle contraction is transmitted directly from the connective tissue harness to the tendons, which subsequently exert force on the bone at the attachment site. These forces can range from 20 to 50 newtons per cm² of cross-sectional area, often surpassing the tolerance of the muscle fibers themselves.
- Sarcolemma: The sarcolemma is a thin, elastic membrane situated beneath the endomysium and enveloping each muscle fiber. It contains a plasma membrane (plasmalemma) and a basement membrane. The plasma membrane, a bilayer lipid structure, plays a crucial role in conducting electrochemical waves during depolarization and insulating muscle fibers from one another during this process. The basement membrane contains proteins and collagen fibrils that merge with the collagenous fibers of the tendon’s outer covering.
- Satellite Cells: Satellite cells, located between the basement and plasma membranes, are myogenic stem cells. They are usually quiescent but can become active in processes such as regenerative cellular growth, adaptations to exercise training, and recovery from injuries. Incorporating satellite cell nuclei into existing muscle fibers is believed to contribute to exercise-induced muscle fiber hypertrophy.
- Sarcoplasm: The sarcoplasm, the aqueous protoplasm of muscle fibers, contains various components, including enzymes, fat and glycogen particles, nuclei, mitochondria, and specialized organelles. Approximately 250 nuclei are present per millimeter of fiber length.
- Sarcoplasmic Reticulum: The sarcoplasmic reticulum forms an extensive, latticelike network of tubular channels and vesicles. This specialized system provides structural support to the muscle cell and facilitates the rapid spread of depolarization waves from the fiber’s outer surface to its interior, initiating muscle action. The sarcoplasmic reticulum also regulates calcium ion concentrations within muscle fibers.
Chemical Composition
The chemical composition of skeletal muscle includes:
- Water, which makes up approximately 75% of muscle mass.
- Protein, comprising about 20% of muscle composition.
- A remaining 5% consisting of salts, high-energy phosphates, urea, lactate, minerals (calcium, magnesium, phosphorus), various enzymes, sodium, potassium, chloride ions, amino acids, fats, and carbohydrates.
- The most abundant muscle proteins are myosin (about 60% of muscle protein), actin, and tropomyosin.
- Each 100 grams of muscle tissue contains approximately 700 milligrams of myoglobin, an oxygen-binding protein.
This comprehensive analysis sheds light on the intricate structure and composition of skeletal muscles, providing valuable insights into their functionality and adaptability in response to various physiological demands.
Blood Supply
Muscles are endowed with a rich vascular supply, with arteries and veins running parallel to individual muscle fibers. These vessels branch into arterioles, capillaries, and venules, forming an extensive network within and around the endomysium. This intricate vascular branching ensures that each muscle fiber receives an ample supply of oxygenated blood from the arterial system, allowing for the rapid removal of carbon dioxide through the venous circulation.
During the most intense exercise, such as that undertaken by elite endurance athletes, the muscle’s demand for oxygen undergoes a remarkable surge. Oxygen consumption in the muscle can increase by nearly 70 times, reaching approximately 11 mL per 100 g of muscle per minute or a total muscle oxygen consumption (V O2) of 3400 mL per minute. To meet this heightened oxygen demand, the local vascular bed orchestrates the delivery of large quantities of blood through active tissues.
In rhythmic activities like running, swimming, or cycling, blood flow distribution exhibits fluctuations. It decreases during the muscle’s contraction phase and increases during relaxation, creating a “milking action” that propels blood through the muscles and back to the heart. The rapid dilation of previously dormant capillaries complements this pulsatile blood flow.
In fact, between 200 and 500 capillaries are responsible for delivering blood to each square millimeter of active muscle cross-section, with up to four capillaries directly contacting each muscle fiber. This vascular adaptation becomes even more pronounced in endurance athletes, with five to seven capillaries surrounding each fiber. This augmentation ensures more significant local blood flow and adequate tissue oxygenation during times of heightened demand.
However, muscle blood flow during strenuous activities involving maximal effort presents a somewhat different scenario. When a muscle generates approximately 60% of its force-generating capacity for an extended period, the elevated intramuscular pressure can momentarily obstruct local blood flow during contraction. In such cases, the muscle relies primarily on intramuscular high-energy phosphates and glycolytic anaerobic reactions as the main energy sources to sustain its efforts.
Capillarization
Enhanced capillary-to-muscle fiber ratios in trained muscles play a pivotal role in improving exercise capacity, particularly in endurance training. This augmentation of capillary microcirculation serves several vital purposes:
- Heat and Metabolic Byproduct Removal: An increased capillary network accelerates the removal of heat and metabolic waste products from active muscle tissues.
- Nutrient and Hormone Delivery: It facilitates the rapid delivery of oxygen, essential nutrients, and hormones to the muscle fibers.
- Improved Oxygenation: The enhanced capillary network ensures that muscles receive a consistent supply of oxygen, even during strenuous activities, enhancing overall endurance and performance.
Electron microscopy reveals that the total number of capillaries per muscle, and capillaries per square millimeter of muscle tissue, averages about 40% higher in endurance-trained athletes compared to their untrained counterparts. This nearly matches the 41% difference in V O2max (maximum oxygen consumption) between the two groups. Additionally, there is a positive association between V O2max and the average number of muscle capillaries.
This enhanced vascularization on the capillary level is particularly advantageous during exercise that demands a high level of steady-rate aerobic metabolism. Intense aerobic training stimulates capillary development through vascular stretch and shear stress on the vessel walls, facilitating increased blood flow during exercise.
The Sarcomere
The distinctive striated appearance of skeletal muscle fibers under low magnification arises from the alternating light and dark bands running along their length. These bands correspond to different structural components within a myofibril, with the I band representing the lighter area and the A band the darker region. The Z line bisects the I band and adheres to the sarcolemma, providing structural stability.
These bands exhibit specific optical properties. Light passing through the I band travels at the same velocity in all directions, making it isotropic. In contrast, light passing through the A band does not scatter equally in all directions, rendering it anisotropic. The term “Z” originates from the German word “zwischenscheibe,” meaning “between,” while “M” (mittelscheibe) signifies “middle,” and “H” (hellerscheibe) denotes “a clear disk or zone.”
The sarcomere, the fundamental repeating unit between two Z lines, represents the functional unit of a muscle fiber. Within a given muscle fiber, sarcomeres are arranged in series, with their filaments running parallel to each other. In its resting state, the average length of a sarcomere is approximately 2.5 micrometers. Consequently, a myofibril measuring 15 millimeters in length comprises about 6000 sarcomeres connected end to end. The length of the sarcomere plays a pivotal role in determining a muscle’s functional properties.
The arrangement of thin actin and thicker myosin proteins within the sarcomere creates an interdigitating overlap between the two filaments. The center of the A band contains the H zone, a region of lower optical density due to the absence of actin filaments. The M band bisects the central portion of the H zone, marking the sarcomere’s center. This M band consists of protein structures that support the organization of myosin filaments.
Muscle Fiber Alignment
The alignment of muscle fibers relative to the long axis of a muscle has a significant impact on its force- and power-generating capabilities. Two primary fiber alignment patterns exist:
- Fusiform Fibers: These spindle-shaped fibers run parallel to the muscle’s long axis, as seen in muscles like the biceps brachii. They taper at their tendinous attachments.
- Pennate Fibers: These fan-shaped fibers have fasciculi (fiber bundles) that lie at an oblique angle, with a pennation angle that can vary up to 30 degrees. Muscles such as the soleus exhibit a pennation angle of around 25 degrees, whereas the vastus medialis has a 5-degree angle, and the sartorius muscle lacks any pennation angle.
The degree of pennation significantly influences the number of sarcomeres per cross-sectional muscle area. Pennate muscles can accommodate a larger number of sarcomeres within a given muscle volume, allowing them to generate more force and power despite having relatively short individual fibers. This ability to pack numerous fibers into a smaller cross-sectional area is known as the physiologic cross-sectional area (PCSA).
Fusiform muscles, on the other hand, lack pennation, so their fiber cross-sectional area represents the true anatomic cross-section. Pennate muscles with their complex arrangement of connective tissue, tendons, and shorter fibers create a larger cross-sectional area than fusiform muscles. Muscle pennation, specifically larger pennation angles, results in a substantial increase in the ability to pack fibers efficiently into a smaller space. This is why pennate muscles tend to generate considerable power.
Complex Fusiform Arrangement
In addition to simple fusiform and pennate muscle fiber arrangements, there is another unique structural adaptation known as the complex parallel muscle or series-fibered muscle arrangement. In this configuration, individual muscle fibers run parallel to the muscle’s line of pull, similar to the simple fusiform arrangement. However, what distinguishes the complex parallel arrangement is that these muscle fibers do not extend the entire length of the muscle. Instead, they terminate within the muscle’s midbelly and gradually taper to interact with the surrounding connective tissue matrix and neighboring muscle fibers.
This structural specialization allows for the parallel packing of relatively short muscle fibers within a longer muscle. For example, the sartorius muscle, which is approximately 50 centimeters long, exhibits this complex parallel arrangement. Within this muscle, individual fibers terminate at various points along the muscle’s length, creating lateral tension through connective tissue into the tendon or through interactions with adjacent and series fibers.
Fiber Length–Muscle Length Ratio
The ratio of individual muscle fiber length to the total length of a muscle typically falls within the range of 0.2 to 0.6. This means that individual muscle fibers, even in the longest muscles such as those in the upper and lower limbs, remain significantly shorter than the overall length of the muscle.
This architectural variability among muscles leads to functional differences. Quadriceps muscles, with their shorter fibers and higher PCSA, are capable of generating approximately 50% more force compared to the hamstrings. Hamstrings, on the other hand, are designed for rapid shortening and are more susceptible to tearing during activities like sprint running, where there can be an abrupt force output imbalance between the quadriceps and hamstrings. This imbalance may arise from a strength deficit between the two muscle groups, making targeted exercise training to improve the hamstring-to-quadriceps strength ratio an essential component of functional rehabilitation for such deficits.
ACTIN–MYOSIN ORIENTATION
Muscle contraction is a complex process that relies on the precise arrangement of actin and myosin filaments within muscle fibers. This article explores the intricate orientation of these filaments, the structural composition of myosin and actin, and the pivotal roles played by regulatory proteins in the contraction and relaxation of muscles.
Arrangement of Actin and Myosin Filaments
In a muscle fiber, the arrangement of myosin filaments alongside actin filaments is a fundamental aspect of muscle function. Even at rest, the sarcomere, which is the functional unit of muscle contraction, exhibits a specific actin–myosin orientation.
Myosin filaments are intricate structures composed of bundles of molecules with polypeptide tails and globular heads. In contrast, actin filaments consist of two twisted chains of monomers held together by tropomyosin polypeptide chains. Within a sarcomere at resting length, six relatively slender actin filaments, each approximately 50 Å in diameter and 1 micron long, encircle a thicker myosin filament measuring around 150 Å in diameter and 1.5 microns long. This highly organized configuration showcases the remarkable precision of muscle fiber organization.
To provide context, a myofibril with a 1-micron diameter contains approximately 450 thick filaments at the center of the sarcomere and 900 thin filaments at each end. Expanding further, a muscle fiber with a diameter of 100 microns and a length of 1 centimeter contains approximately 8,000 myofibrils, with each myofibril averaging 4,500 sarcomeres. In a single muscle fiber, this astonishing arrangement results in roughly 16 billion thick filaments and 64 billion thin filaments.
Globular Myosin Heads and Crossbridges
A striking feature of muscle contraction is the involvement of globular myosin heads that project perpendicularly from the myosin filaments, resembling lollipops. These myosin heads form both structural and functional links between myofilaments. The myosin heads attach themselves to the thinner double-twisted actin strands, creating connections that are vital for muscle contraction.
What sets the myosin heads apart is their orientation at the ends of the thick filament, where two heads point in opposite directions. The activation of these myosin heads through ATP hydrolysis places them in an optimal position to bind to the active sites on actin filaments. This activation initiates the pulling of the thin filaments and Z lines toward the center of the sarcomere, setting the stage for muscle contraction.
Regulation by Tropomyosin and Troponin
Two essential regulatory proteins within the actin filaments are tropomyosin and troponin. Tropomyosin is distributed along the length of the actin filament, fitting snugly into a groove formed by the double helix. It plays a pivotal role in inhibiting the interaction (coupling) between actin and myosin, thus preventing their permanent bonding.
Troponin, composed of three subunits (troponin I, T, and C), is embedded at regular intervals along the actin strands. Troponin exhibits a high affinity for calcium ions (Ca2+), a mineral that plays a crucial role in muscle action and fatigue. When Ca2+ binds to troponin, it triggers a conformational change in troponin molecules, exerting a “tugging” effect on tropomyosin protein strands. Consequently, tropomyosin shifts deeper into the groove between the actin strands, “uncovering” the active sites on actin. This uncovering allows muscle action to proceed. Muscle fatigue often arises from significant reductions in Ca2+ concentration in the transverse tubules during intense exercise, combined with intrinsic changes in the contractile apparatus and sarcoplasmic reticulum function.
The M Band and Cytoskeletal Proteins
The M band, a structural component within the sarcomere, consists of proteins that maintain the orientation of myosin filaments. These proteins are transversely and longitudinally oriented and connect with six adjacent myosin filaments in a hexagonal pattern, contributing to the structural integrity of the sarcomere.
An intriguing area of research in muscle biology focuses on the study of cytoskeletal proteins and structures that form an intermediate intracellular filament system. These cytoskeletal elements provide structural integrity to inactive muscle cells, facilitate the transmission of lateral forces between adjacent sarcomeres during muscle action, and establish connections with the cell’s surface membrane. Further understanding of the role of the cytoskeleton and its interactions with myofibrillar lattice structures promises to enhance our comprehension of muscle action, including processes related to muscle injury, repair, and overload.
Intracellular Tubule Systems
The muscle fiber contains a complex tubule system. The lateral ends of each tubule channel terminate in saclike vesicles responsible for storing calcium ions (Ca2+). Another network of tubules, referred to as the transverse tubule system or T-tubule system, runs perpendicular to the myofibrils. These T-tubules are situated between the most lateral parts of two sarcoplasmic channels, with vesicles from these structures abutting the T-tubules. The term “triad” describes the repeating pattern of two vesicles and a T-tubule present at each Z-line region. Each sarcomere contains two triads, and this pattern is repeated regularly along the myofibril’s length.
The T tubules pass through the fiber and open externally from the inside of the muscle cell. The triad and T-tubule system function as a microtransportation network, spreading the action potential (wave of depolarization) from the fibers’ outer membrane inward to deeper cell regions. This propagation of the action potential stimulates the triad sacs to release Ca2+, which diffuses a short distance to “activate” the actin filaments. Muscle action commences as myosin filament crossbridges momentarily attach to active sites on the actin filaments. When electrical excitation ceases, Ca2+ concentration in the cytoplasm decreases, leading to muscle relaxation.
To a certain extent, the propagation of an action potential (and the mitigation of fatigue during exercise) depends on maintaining steep gradients of sodium (Na+) and potassium (K+) ions across the sarcolemma. A reduction in the chemical gradients of these electrolytes, resulting from decreased Na+/K+ pump activity, can severely affect muscle fiber excitability and, consequently, the contractile performance of active muscles.
Chemical and Mechanical Events During Muscle Action and Relaxation
The intricate processes that underlie muscle action and relaxation have been unraveled through the use of electron microscopy, X-ray diffraction, and biochemical methods. These techniques have provided valuable insights and testable hypotheses about the chemical and mechanical events that occur during muscle activation and relaxation.
Exploring Muscle Cytoskeletal Proteins and Intracellular Tubule Systems
Muscle contraction is a remarkable interplay of biochemical, physiological, and mechanical processes. A fascinating realm of study in the field of muscle biology involves delving into cytoskeletal proteins and intracellular tubule systems. These components play pivotal roles in maintaining muscle integrity, transmitting lateral forces during contraction, and ensuring efficient communication between muscle cells.
Cytoskeletal Proteins: The Intricate Muscle Framework
At the heart of this captivating area of research lies the cytoskeleton of muscle cells. This intricate network of proteins and structures serves as an intermediate intracellular filament system. The cytoskeleton offers a multifaceted set of functions that profoundly impact muscle physiology:
- Structural Integrity: In its inactive state, the cytoskeleton provides essential structural integrity to muscle cells. It acts like a scaffold, lending support to the cell even when it’s not actively contracting. This structural stability is crucial for maintaining the overall shape and function of the muscle.
- Lateral Force Transmission: During muscle action, the cytoskeleton plays a crucial role in transmitting lateral forces. As muscles contract, forces are generated along their length. The cytoskeleton aids in distributing these forces to adjacent sarcomeres—a structural unit of muscle tissue. This inter-sarcomere interaction is vital for coordinated muscle contraction.
- Connections to the Cell’s Surface Membrane: Muscle cells must communicate with the cell’s surface membrane, known as the sarcolemma, to initiate and propagate action potentials. The cytoskeleton forms connections that facilitate this communication, allowing for the rapid transmission of electrical signals.
A deeper understanding of the diverse proteins and the lattice-like structure of the myofibrils that make up the muscle fiber should significantly advance our comprehension of muscle function. This enhanced understanding can shed light on critical aspects of muscle physiology, such as injury, repair, and overload responses.
Intracellular Tubule Systems:
- Sarcoplasmic Reticulum: The lateral ends of each tubule channel in this system terminate in saclike vesicles that function as calcium (Ca2+) storage units. Calcium ions are essential for muscle contraction. The sarcoplasmic reticulum regulates the release of Ca2+ into the cytoplasm when muscle action is initiated.
- Transverse Tubules (T-Tubules): Running perpendicular to the myofibrils, the T-tubules traverse the muscle fiber. They are situated between the most lateral parts of two sarcoplasmic channels, with vesicles from these structures abutting the T-tubules. This arrangement forms a repeating pattern known as a “triad,” which consists of two vesicles and a T-tubule located at each Z-line region. Each sarcomere within the muscle contains two such triads, and this pattern repeats regularly along the myofibril’s length.
The T-tubule system, along with the triads, serves as a microtransportation network within the muscle fiber. Its primary function is to rapidly spread the action potential, which is a wave of depolarization, from the outer membrane of the muscle fiber to deeper cell regions. This action potential stimulates the release of calcium ions from the sarcoplasmic reticulum into the cytoplasm, which is a crucial step in muscle contraction.
Initiation of Muscle Action and Regulation
The initiation of muscle action is a complex process that involves the interaction of various structures within the muscle fiber. When the action potential reaches the T-tubules, it triggers the release of calcium ions from the sarcoplasmic reticulum. These calcium ions then diffuse a short distance, where they “activate” the actin filaments.
Muscle action begins as myosin filament crossbridges momentarily attach to active sites on the actin filaments. These crossbridges exert force and generate movement, leading to muscle contraction. When the electrical excitation ceases, calcium ion concentration in the cytoplasm decreases, initiating muscle relaxation.
To maintain the proper propagation of an action potential and counteract fatigue during exercise, it is essential to sustain steep gradients of sodium (Na+) and potassium (K+) ions across the sarcolemma. A decrease in these chemical gradients, resulting from reduced activity of the sodium-potassium pump (Na+/K+ pump), can significantly impact the excitability of muscle fibers and, consequently, the contractile performance of active muscles.
Unveiling the Chemical and Mechanical Dynamics of Muscle Action and Relaxation
The world of muscle biology is a realm of intricate chemical and mechanical events that orchestrate the marvel of muscle action and relaxation. Through cutting-edge techniques like electron microscopy, X-ray diffraction, and advanced biochemical methods, scientists have unveiled many secrets of cellular structure and kinetics. These revelations have led to the development of testable hypotheses and, in particular, have shed light on the processes underpinning muscle activation and relaxation.
The Sliding-Filament Model:
One of the most influential theories in muscle biology is the sliding-filament model, which was proposed around six decades ago by two British biologists, Hugh Huxley and Sir Andrew Fielding Huxley. This groundbreaking model has remained a cornerstone in the field, providing a coherent framework for comprehending the intricate molecular movements that underlie muscle action. Sir Andrew Fielding Huxley, in particular, was awarded the Nobel Prize in Physiology or Medicine in 1963 for his work on ionic mechanisms involved in excitation and inhibition in the peripheral and central portions of the nerve cell membrane.
At its core, the sliding-filament model presents a compelling explanation for muscle contraction. It posits that when a muscle contracts, neither the thick nor the thin filaments change in length; instead, they slide past each other. This elegant concept serves as the foundation for understanding the mechanical basis of muscle shortening and lengthening. Here are the key components of this model:
- Filament Sliding: The model proposes that the thick myosin filaments and the thin actin filaments within the muscle slide past each other without any alteration in their individual lengths. This coordinated sliding motion is the primary mechanism responsible for muscle contraction and relaxation.
- Role of Myosin Crossbridges: Central to this model are myosin crossbridges. These molecular structures cyclically attach to, rotate, and detach from the actin filaments, fueled by the energy derived from the hydrolysis of ATP (adenosine triphosphate). This energy-driven interaction serves as the molecular motor that drives muscle fiber shortening.
- Conformational Changes: As myosin crossbridges interact with actin filaments, they undergo significant conformational changes. These alterations in molecular shape result in a shift in the relative sizes of various zones and bands within the sarcomere, the structural unit of muscle tissue.
- Structural Rearrangements: During muscle shortening, the most prominent structural rearrangement occurs in the region of the I band. This band, representing the region containing only thin actin filaments, decreases in width as the Z bands (the ends of sarcomeres) are pulled toward the center of each sarcomere. Conversely, during muscle lengthening or relaxation, the I band widens as the actin filaments move away from the center.
- Isometric vs. Eccentric Action: Muscle action can manifest in different ways. In isometric muscle action, the muscle generates force, but the length of the muscle fibers remains unchanged. In this scenario, the relative spacing of the I band and the A band (the region containing thick myosin filaments) remains constant. However, in eccentric action, the muscle fibers lengthen as force is generated, resulting in the widening of the A band.
Unlocking the Mechanical Intricacies of Crossbridges in Muscle Action
Within the realm of muscle biology, the mechanical actions of crossbridges stand as a pivotal facet of the intricate symphony that orchestrates muscle function. Myosin, a key player in this intricate drama, assumes both an enzymatic and structural role in muscle action. At the heart of this process is the globular head of the myosin crossbridge, which houses an actin-activated ATPase in its actin-binding site. This head serves as the powerhouse, driving the mechanical power stroke that enables the actin and myosin filaments to glide past each other in a choreographed manner.
The motion of crossbridges is far from synchronous; instead, it resembles the rhythmic to-and-fro movement of oars slicing through water. This asynchrony in crossbridge movement is crucial for the generation of finely graded, smoothly modulated muscle movements and force outputs during shortening. While about 50% of the crossbridges engage with actin filaments to form the contractile actomyosin complex at any given moment, the rest continue their oscillatory cycles in a highly coordinated fashion.
To appreciate the subtlety of this intricate dance, consider it akin to a person climbing a rope. In this analogy, the arms and legs of the climber represent the crossbridges. Progress in climbing is achieved through a sequence of movements: reaching with the arms, grasping, pulling, releasing while extending the legs, and then repeating this cyclic pattern as the climber ascends. Similarly, crossbridges undergo a series of individual cycles, each contributing only a modest longitudinal displacement to the overall sliding action of the filaments.
To delve deeper into the mechanical properties of actin and myosin interactions, researchers have devised several innovative techniques:
- Microneedles: By placing a glass needle in contact with myosin molecules and an actin filament, scientists can record the mechanical movements of these molecules. This method helps deduce the forces generated by myosin heads as they glide along the actin strand.
- Optical Tweezers: This technique combines powerful laser technology with microscopy to isolate individual molecules and meticulously measure their movement, one molecule at a time.
- Atomic Force Microscope: By interfacing a probe with actin and myosin molecules attached with a specialized microscope, researchers gain quantitative insights into actin-myosin interaction.
- Fluorescent Probes: These light-emitting probes are instrumental in quantifying the kinetics of molecular binding and release between myosin and actin, shedding light on the energy release when ATP is degraded to ADP and inorganic phosphate.
The mechanistic revelations from these techniques have uncovered that myosin generates a force ranging from 1 to 10 piconewtons (pN) over a 5-millisecond interval, with myosin movement spanning 1 to 20 nanometers (nm). These findings provide essential insights into the delicate dance of molecular forces that underlie muscle action.
Isometric Tension and the Length-Tension Curve
In the realm of muscle physiology, the concept of isometric tension and the length-tension curve play a pivotal role in understanding muscle function. The length-tension curve illustrates the relationship between the length of a sarcomere (the basic contractile unit of muscle) and the amount of tension it can generate.
Researchers, about 45 years ago, conducted pioneering experiments to construct the length-tension curve. They electrically stimulated a single frog muscle fiber and meticulously plotted the maximum tension output at various sarcomere lengths. The curve revealed some intriguing insights:
- The point of maximum tension occurred at a sarcomere length between 2.0 and 2.25 micrometers (µm), representing the region of optimal actin and myosin filament interaction.
- This region of maximal tension corresponds precisely to the width of the sarcomere where no change occurs in actin-myosin interaction.
- When the sarcomere stretched beyond 2.2 µm, there was a decline in peak tension due to reduced overlap between actin and myosin filaments, resulting in diminished crossbridge interaction and reduced active tension development.
- Beyond a sarcomere length of 3.65 µm and above, the muscle fiber failed to develop tension, as crossbridge interaction became impossible.
In Vivo Insights into Sarcomere Length in Human Muscle
Translating these principles to the realm of human muscle in vivo, scientists have conducted elegant experiments to determine the range over which sarcomeres in intact human muscle operate on their length-tension curve. This endeavor involved studying patients undergoing surgery to correct chronic lateral epicondylitis (commonly known as “tennis elbow”).
Through a sophisticated intraoperative procedure involving a helium-neon laser to measure sarcomere length, researchers explored the length-tension characteristics of the extensor carpi radialis brevis muscle (ECRB) during various wrist positions. Their findings revealed the intrinsic relationship between sarcomere length and muscle fiber force capacity. These experiments further emphasized the importance of the length-tension curve in understanding the functional properties of human muscle.
Unlocking the Molecular Symphony: Actin, Myosin, and ATP in Muscle Action
The intricate world of muscle action is governed by the harmonious interplay of protein filaments, notably actin and myosin, orchestrated by the energy currency of cells, adenosine triphosphate (ATP). This dynamic interaction and movement of protein filaments are the fundamental processes underlying muscle contraction and relaxation, providing us with the remarkable ability to move and exert force.
Oscillatory Movements of Myosin Crossbridges Central to the process of muscle action is the continuous, rhythmic dance of myosin crossbridges. These crossbridges perpetually undergo oscillatory movements, combining, detaching, and recombining with various sites along the actin strands. Whether in dynamic or static muscle actions, this perpetual movement of myosin crossbridges is essential.
The Role of ATP: A Molecular Key The detachment of myosin crossbridges from actin filaments hinges on the arrival of ATP molecules, which join the actomyosin complex, instigating a critical chemical reaction. In this reaction, actomyosin dissociates into its constituent parts, forming a new active site for future interaction:
Actin + Myosin ATPase : Actomyosin + ATPase
The magic happens when ATP undergoes hydrolysis, a process that transduces energy from ATP into mechanical force as adenosine diphosphate (ADP) and inorganic phosphate are generated. This intricate process begins as one of the active sites on the globular head of the myosin crossbridge binds to an actin reactive site. Meanwhile, the other active site on myosin serves as an actin-activated enzyme known as myofibrillar adenosine triphosphatase (myosin ATPase). This enzyme is responsible for splitting ATP to release the energy required for muscle action. Notably, the rate of ATP splitting is relatively sluggish when myosin and actin remain apart. However, when they join, myosin ATPase reaction rates significantly accelerate, igniting the crossbridges into action.
The Energetic Dance of Crossbridges:
The energy derived from the splitting of ATP into ADP and inorganic phosphate fuels the rhythmic oscillation of crossbridges, causing them to vibrate like finely-tuned strings in a musical instrument. This energetic transfer sparks a conformational change in the globular head of myosin, aligning it to interact with the appropriate actin molecule.
The Cocked Spring Mechanism:
Before muscle action is initiated, the elongated, pear-shaped, and flexible myosin head undergoes a remarkable transformation. It bends around the energy-laden ATP molecule and “cocks” like a tightly wound spring. The myosin head, in this primed state, is now ready to interact with the adjacent actin filament. The subsequent splitting of a phosphate from ATP releases its stored mechanical energy, forcing a sliding motion between actin and myosin, the very motion that generates the remarkable muscle tension we observe during contraction. This coordinated interplay between actin and myosin filaments is akin to the gears of a well-oiled machine working in unison, allowing the actin and myosin filaments to glide past each other at speeds of up to 15 micrometers per second.
Initiating Muscle Action:
The onset of muscle action is initiated by a physiological mechanism known as excitation–contraction coupling. This intricate process is akin to the conductor raising the baton before a grand musical performance, signaling the commencement of a symphony of events within the muscle.
The Role of Calcium Ions (Ca²⁺):
Central to excitation–contraction coupling is the role of calcium ions (Ca²⁺). Inside a resting muscle fiber, the concentration of Ca²⁺ remains relatively low compared to the extracellular fluid that bathes the cell. However, when the muscle is stimulated, there is an immediate, albeit small, increase in intracellular Ca²⁺ levels, preceding any noticeable contractile activity. This surge in Ca²⁺ concentration occurs as a result of the action potential at the transverse tubules, which triggers the release of Ca²⁺ from the lateral sacs of the sarcoplasmic reticulum, a specialized structure within muscle cells.
Unlocking the Contractile Machinery:
The surge in intracellular Ca²⁺ levels initiates a cascade of events that essentially “unlocks” the contractile machinery. In a resting muscle, the inhibitory action of a protein called troponin prevents the interaction between actin and myosin. However, when Ca²⁺ binds with troponin and other proteins within the actin filaments, this inhibition rapidly dissipates, allowing the actin and myosin to come together. This is the critical moment when the muscle is primed for action.
Coupling and Uncoupling:
As the actin and myosin filaments interact, the myosin ATPase enzyme comes into play, splitting ATP and generating the energy required for the crossbridges to move and produce muscle tension. This dance of coupling and uncoupling continues as long as the concentration of Ca²⁺ remains high enough to inhibit the troponin-tropomyosin system. Once neural stimulation ceases, Ca²⁺ is efficiently transported back into the lateral sacs of the sarcoplasmic reticulum. This process swiftly restores the inhibitory action of troponin-tropomyosin, keeping actin and myosin apart. As long as ATP concentration remains adequate, the muscle fibers remain relaxed, awaiting the next cue for action.
The Stiffening Embrace of Rigor Mortis:
In the aftermath of death, muscle fibers undergo a phenomenon known as rigor mortis, where they stiffen and become rigid. This occurs because, in the absence of ATP, the myosin crossbridges and actin remain attached and do not separate. Rigor mortis serves as a stark reminder of the fundamental role ATP plays in controlling muscle contraction.
In essence, the complex interplay between actin, myosin, calcium ions, and ATP in muscle action is a testament to the extraordinary intricacy of our bodies. This molecular symphony unfolds with precision, allowing us to perform the countless movements and feats that define our physical capabilities.
Relaxation: The Calm After the Storm:
After the culmination of a muscular performance, during which intricate molecular machinery orchestrated the dance of actin and myosin, a period of relaxation ensues. This phase is marked by the cessation of muscle stimulation and the return of various molecular components to their resting states, allowing the muscle fibers to unwind and prepare for the next act.
Ceasing the Flow of Calcium (Ca²⁺):
The relaxation process kicks off with the halt of calcium ion (Ca²⁺) flow. As muscle stimulation ceases, Ca²⁺ movement ceases as well, and troponin, a regulatory protein, is free to inhibit the interaction between actin and myosin. This inhibition is vital for preventing unwanted muscle contractions.
The Sarcoplasmic Reticulum’s Role:
The intricate process of recovery involves the active pumping of Ca²⁺ ions back into the sarcoplasmic reticulum. Within this specialized structure, Ca²⁺ ions are concentrated within the lateral vesicles. This serves two critical purposes: first, it prevents any mechanical link between the myosin crossbridges and actin filaments, ensuring that the muscle remains relaxed, and second, it inhibits myosin ATPase activity, effectively curbing ATP splitting.
Muscle Relaxation:
Muscle relaxation represents a return to equilibrium, where the actin and myosin filaments revert to their original states. This return to baseline molecular configurations is essential to ensure that the muscle is ready for subsequent contractions.
The Sequence of Muscle Action:
The orchestration of muscle action can be likened to a meticulously choreographed ballet, where each step and movement contributes to the graceful performance. Let’s break down the key events in the sequence of muscle action, elucidating how they harmonize to create motion:
Step 1: Initiation of the Action Potential: The performance begins with the initiation of an action potential by the motor nerve. This electrical impulse prompts small vesicles within the terminal axon to release a neurotransmitter called acetylcholine (ACh). ACh diffuses across the synaptic cleft and attaches to specialized ACh receptors on the sarcolemma, the muscle cell membrane. This binding is akin to a conductor signaling the first notes of the symphony.
Step 2: Depolarization of Transverse Tubules: The muscle action potential propagates across the sarcolemma, depolarizing the transverse tubules (T-tubules) at the A–I junction within the sarcomere, the basic contractile unit of a muscle fiber.
Step 3: Ca²⁺ Release: The depolarization of the T-tubule system triggers the release of Ca²⁺ ions from the lateral sacs (terminal cisternae) of the sarcoplasmic reticulum. This release of Ca²⁺ is akin to unlocking the gates, allowing the subsequent molecular events to unfold.
Step 4: Ca²⁺ Binding to Troponin–Tropomyosin Complex: Ca²⁺ ions bind to troponin–tropomyosin complexes within the actin filaments. This binding releases the inhibition that was previously preventing actin from binding with myosin, setting the stage for muscle contraction.
Step 5: Actin-Myosin Interaction and ATP Splitting: As the performance intensifies, actin combines with myosin-ATP. Actin also activates the enzyme myosin ATPase, which catalyzes the splitting of ATP. The energy generated from this reaction powers the movement of myosin crossbridges, creating tension in the muscle fibers.
Step 6: ATP Binding and Crossbridge Dissociation: To allow for the muscle to relax and prepare for the next act, ATP binds to the myosin crossbridge. This binding breaks the bond between actin and myosin, allowing the crossbridge to dissociate from actin. Consequently, the thick and thin filaments slide past each other, and the muscle shortens or lengthens accordingly.
Step 7: Maintaining Crossbridge Activation: The activation of crossbridges persists as long as the intracellular Ca²⁺ concentration remains sufficiently high, primarily due to membrane depolarization, which inhibits the troponin–tropomyosin system.
Step 8: Rapid Ca²⁺ Removal: As the curtain falls on the performance, intracellular Ca²⁺ concentration rapidly decreases. This is achieved through active transport, which requires ATP hydrolysis. Ca²⁺ ions are actively moved back into the lateral sacs of the sarcoplasmic reticulum.
Step 9: Restoring Inhibition: With Ca²⁺ ions removed, the inhibitory action of troponin–tropomyosin is restored. In the presence of ATP, actin and myosin remain in a dissociated, relaxed state, ready to embark on another performance.
In this intricately coordinated sequence of events, the molecular symphony of muscle action unfolds with remarkable precision, allowing our bodies to execute the myriad of movements that define our physical abilities. Whether it’s the graceful grace of a dancer or the powerful prowess of an athlete, it’s the orchestrated interplay of actin, myosin, calcium ions, and ATP that makes it all possible.
Muscle Fiber Types: The Mosaic of Muscle Composition
Within the intricate tapestry of skeletal muscle, there exists a rich diversity of muscle fibers, each with its unique set of metabolic and contractile properties. Rather than a uniform assembly, skeletal muscle is composed of two primary types of muscle fibers that differ in their mechanisms for producing ATP, their motor neuron innervation, and the type of myosin heavy chain they express. The proportions of these muscle fiber types can vary significantly between muscles and among individuals.
Distinguishing Fiber Types through Myosin Heavy Chains:
One of the key techniques for classifying muscle fiber types involves assessing the heavy chain of the myosin molecule, which exists in three distinct isoforms. This assessment involves evaluating how sensitive a fiber is to changes in pH regarding the enzyme myosin ATPase, a measure of myosin phenotype. The unique characteristics of this enzyme dictate the rate of ATP hydrolysis in the myosin heavy chain region, ultimately influencing the velocity of sarcomere shortening.
Fast-twitch fibers, also known as Type II fibers, exhibit distinct characteristics that set them apart:
- High Electrochemical Transmission: Fast-twitch fibers are adept at transmitting action potentials rapidly.
- Elevated Myosin ATPase Activity: These fibers possess high myosin ATPase activity, contributing to their rapid contractile abilities.
- Efficient Ca²⁺ Handling: Fast-twitch fibers exhibit swift release and uptake of calcium ions, facilitated by an efficient sarcoplasmic reticulum.
- Rapid Crossbridge Turnover: These fibers boast a high rate of crossbridge turnover, which is pivotal for quick, powerful muscle actions.
Fast-twitch fibers harness these attributes to generate energy rapidly, enabling swift and forceful muscle contractions. Their intrinsic speed of shortening and tension development outpaces that of slow-twitch fibers by three to five times, making them indispensable for explosive movements and anaerobic activities such as sprinting and stop-and-go sports like basketball, soccer, lacrosse, or field hockey. These sports rely heavily on anaerobic energy metabolism, which fast-twitch fibers excel at.
Subtypes of Fast-Twitch (Type II) Fibers:
Within the realm of fast-twitch fibers, there exist three primary subtypes:
- Type IIa: These fibers feature rapid shortening speed and possess a moderately developed capacity for energy transfer from both aerobic and anaerobic sources. They are often referred to as fast–oxidative–glycolytic (FOG) fibers.
- Type IIx: Falling between Type IIa and Type IIb in terms of physiological and metabolic characteristics, Type IIx fibers exhibit a balanced profile of aerobic and anaerobic capacity.
- Type IIb: Also known as true fast-glycolytic (FG) fibers, Type IIb fibers exhibit the highest anaerobic potential and the most rapid shortening velocity.
Recent studies have revealed that human skeletal muscle contains not only Type IIa, Type IIx (previously classified as Type IIb), but also a new Type IIb subtype. These subtypes exhibit variations in their energy pathways, making them suitable for different activities and performance demands.
This intricate mosaic of muscle fiber types showcases the remarkable adaptability and versatility of our skeletal muscles, allowing us to excel in various physical pursuits, from endurance activities to explosive bursts of power. Understanding the nuances of muscle fiber types provides valuable insights for athletes, trainers, and researchers striving to optimize human performance.
Slow-Twitch Fibers (Type I): The Endurance Specialists
Amidst the spectrum of skeletal muscle fibers, slow-twitch fibers, often denoted as Type I fibers, stand out as the stalwarts of endurance. These fibers rely predominantly on the aerobic system for ATP resynthesis, making them exceptionally suited for prolonged physical activities. Here, we delve into the defining characteristics of these endurance specialists:
1. Low Myosin ATPase Activity: Slow-twitch fibers exhibit relatively low myosin ATPase activity, contributing to their unhurried contraction speed.
2. Sluggish Calcium Handling and Shortening Speed: These fibers are characterized by a leisurely pace of calcium handling and sarcomere shortening.
3. Moderate Glycolytic Capacity: Slow-twitch fibers possess a less developed glycolytic capacity compared to their fast-twitch counterparts.
4. Abundance of Mitochondria: With large numbers of mitochondria, slow-twitch fibers bask in a rich supply of these cellular powerhouses. This profusion of mitochondria, combined with iron-containing cytochromes and high myoglobin levels, grants these fibers their distinctive red pigmentation.
Fatigue Resistance and Aerobic Dominance: Slow-twitch fibers shine in the realm of fatigue resistance, thanks to their robust aerobic metabolic machinery. They have earned the moniker “SO” (slow-oxidative) fibers due to their leisurely contraction speed and reliance on oxidative metabolism. These fibers are selectively recruited during aerobic activities, such as long-distance running, cycling, or swimming, where endurance is paramount.
Fueling Endurance: Prolonged, high-intensity aerobic exercise primarily calls upon the services of slow-twitch muscle fibers. Even after a grueling 12-hour workout, the residual glycogen in active muscles is mostly found in the relatively “unused” fast-twitch fibers. Furthermore, slow-twitch fibers determine the magnitude of blood flow through muscle tissue during exercise, receiving the lion’s share.
Classification: In most classifications, slow-twitch fibers are designated as type I, while fast-twitch fibers and their subtypes are labeled as type II. Both slow and fast muscle fiber types contribute to various extents during activities that combine near-maximum aerobic and anaerobic demands, such as middle-distance running, swimming, basketball, field hockey, or soccer.
Genetic Control of Muscle Fiber Type:
The regulation of skeletal muscle fiber types in adult animals, including humans, involves several independent signaling pathways. These pathways include Ras/mitogen-activated protein kinase (MAPK), calcineurin, calcium/calmodulin-dependent protein kinase IV, and peroxisome proliferator-activated receptor-gamma coactivator 1-alpha (PGC-1α). PGC-1α, in particular, plays a pivotal role in promoting mitochondrial biogenesis, mitochondrial fatty acid oxidation, and hepatic gluconeogenesis. It acts as a link between external physiological stimuli and the regulation of muscle fiber type.
This intricate network of signaling pathways not only governs muscle fiber type transformation but also influences metabolic profiles, providing protection against conditions such as insulin resistance and obesity. Other pathways, like the Ras/MAPK signaling pathway, establish connections between motor neurons and signaling systems, facilitating the coupling of excitation and transcription regulation for muscle regeneration.
Additionally, within muscle fibers, physical force generation can release the transcription factor serum response factor (SRF) from the structural muscle protein titin, contributing to muscle growth. These multifaceted pathways underscore the complexity of skeletal muscle fiber type determination and highlight the interplay between genetics, physiology, and exercise adaptation in shaping our muscular composition.
Muscle Fiber Types and Athletic Groups:
The composition of muscle fiber types within the human body can significantly impact athletic performance. Here, we explore the intricate relationship between muscle fiber types and various athletic groups, shedding light on how specific training regimens can influence fiber composition and metabolic capacity.
Baseline Fiber Type Distribution:
On average, individuals of different genders and ages possess roughly 45 to 55% slow-twitch fibers in their arm and leg muscles. Fast-twitch fibers, on the other hand, are typically divided equally between type IIa and type IIb subdivisions. While gender doesn’t seem to influence fiber distribution, there is considerable interindividual variation. Interestingly, the distribution of muscle fiber types tends to remain consistent across an individual’s major muscle groups.
Endurance Athletes: Masters of Slow-Twitch Fibers
Successful endurance athletes, such as long-distance runners and cross-country skiers, exhibit a predominant presence of slow-twitch fibers in the major muscles relevant to their sport. In some cases, these athletes may possess as much as 90 to 95% slow-twitch fibers in muscles like the gastrocnemius of the leg. This high proportion of slow-twitch fibers aligns with their exceptional aerobic and endurance capacities.
Sprint Athletes: The Fast-Twitch Dominance
In stark contrast, elite sprint athletes, like sprinters and powerlifters, demonstrate a prevalence of fast-twitch fibers and generally possess lower aerobic capacities. These athletes rely on the explosive power and rapid force generation enabled by their fast-twitch muscle fibers.
Middle-Distance Athletes: A Balanced Blend
Athletes specializing in middle-distance events tend to strike a balance, with approximately equal percentages of slow-twitch and fast-twitch fibers. This equilibrium allows them to excel in activities that demand both endurance and power.
Beyond Fiber Types: The Holistic Athletic Equation
It’s crucial to note that while muscle fiber composition plays a pivotal role in athletic performance, it’s just one piece of the puzzle. Success in sports hinges on a complex interplay of physiological, biochemical, neurological, and biomechanical factors, with muscle fiber type serving as a critical but not sole determinant.
Adaptations through Training: A Morphological Journey
Endurance athletes often exhibit relatively normal-sized muscle fibers, with a notable propensity for enlargement in their slow-twitch fibers. Conversely, power athletes, like weightlifters, undergo significant hypertrophy, enlarging both fast-twitch and slow-twitch fibers. The fast-twitch fibers of power athletes can exceed those of endurance athletes or sedentary individuals of the same age by up to 45%.
The Gender Factor: Muscle Morphology Differences
Gender differences in muscle morphology are primarily characterized by larger muscle fibers in male athletes and a greater total muscle mass. These disparities contribute to variations in athletic performance between men and women.