Respiratory Physiology
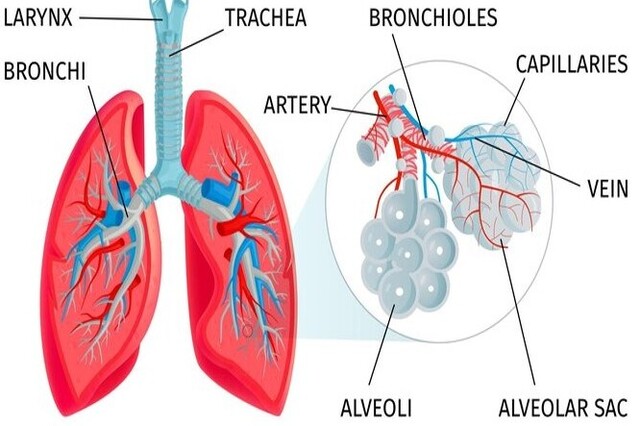
The Respiratory and Cardiovascular Systems:
- The respiratory and cardiovascular systems work together to deliver oxygen to all tissues of the body and remove carbon dioxide.
- This process involves four separate processes:
- Pulmonary ventilation (breathing): movement of air into and out of the lungs.
- Pulmonary diffusion: the exchange of oxygen and carbon dioxide between the lungs and the blood.
- Transport of oxygen and carbon dioxide via the blood.
- Capillary diffusion: the exchange of oxygen and carbon dioxide between the capillary blood and metabolically active tissues.
External Respiration:
- The first two processes, pulmonary ventilation and pulmonary diffusion, are referred to as external respiration.
- External respiration involves moving gases from outside the body into the lungs and then into the blood.
Internal Respiration:
- Internal respiration occurs when blood arrives at the tissues and involves the gas exchange between the blood and the tissues.
- External and internal respiration are linked by the circulatory system.
Pulmonary Ventilation:
- Pulmonary ventilation, or breathing, is the process of moving air into and out of the lungs.
- Air is typically drawn into the lungs through the nose or mouth and travels through various anatomical structures, including the pharynx, larynx, trachea, and bronchial tree.
- The respiratory bronchioles and alveoli are the smallest respiratory units where gas exchange occurs.
- The pleural sacs envelop the lungs, reducing friction during respiratory movements, and are connected to the inner surface of the thoracic cage, allowing the lungs to expand and contract during breathing.
- The anatomy of the lungs, pleural sacs, diaphragm muscle, and thoracic cage determines airflow into and out of the lungs during inspiration and expiration.
The respiratory and cardiovascular systems work in tandem to facilitate the exchange of oxygen and carbon dioxide in the body, with specific processes involved in external and internal respiration. Pulmonary ventilation, or breathing, is a crucial part of this process, involving the movement of air through various anatomical structures in the respiratory system.
Inspiration:
- Inspiration is an active process involving the diaphragm and the external intercostal muscles.
- During inspiration, the external intercostal muscles cause the ribs and sternum to move up and out, while the diaphragm contracts and flattens toward the abdomen.
- These actions expand the thoracic cage in all dimensions, increasing lung volume and reducing intrapulmonary pressure, allowing air to rush into the lungs due to the pressure difference.
Forced Inspiration:
- During forced or labored breathing, as during heavy exercise, other muscles such as scaleni, sternocleidomastoid, and pectorals in the neck and chest assist in raising the ribs further than during regular breathing.
Expiration at Rest:
- At rest, expiration is a passive process involving relaxation of the inspiratory muscles and elastic recoil of lung tissue.
- As the diaphragm and external intercostal muscles relax, the ribs, sternum, and lung tissue return to their resting positions, increasing lung pressure and causing air to be forced out of the lungs.
Forced Expiration:
- During forced breathing, expiration becomes an active process.
- Internal intercostal muscles pull the ribs down, and muscles like the latissimus dorsi and quadratus lumborum can assist in this process.
- Contracting the abdominal muscles increases intra-abdominal pressure, aiding the return of the diaphragm to its domed position and pulling the ribcage down and inward.
Respiratory Pump:
- Changes in intra-abdominal and intrathoracic pressure during forced breathing assist in returning venous blood back to the heart.
- This pressure change is transmitted to the great veins (pulmonary veins and superior and inferior venae cavae) that transport blood back to the heart.
- The changing pressures in the abdomen and thorax create a milking action on the blood in the veins, assisting in its return to the heart.
- This phenomenon is known as the respiratory pump and is essential for maintaining adequate venous return.
Pulmonary Volumes:
- Spirometry is a technique used to measure lung volumes and capacities.
- Tidal volume is the amount of air entering and leaving the lungs with each breath.
- Vital capacity (VC) is the greatest amount of air that can be expired after a maximal inspiration.
- Residual volume (RV) is the amount of air remaining in the lungs after a maximal expiration and cannot be measured with spirometry.
- Total lung capacity (TLC) is the sum of vital capacity and residual volume.
Pulmonary Diffusion:
- Pulmonary diffusion is the exchange of oxygen and carbon dioxide between the alveoli in the lungs and the capillary blood.
- It serves to replenish oxygen in the blood, which is depleted at the tissue level during oxidative energy production.
- It also removes carbon dioxide from returning systemic venous blood.
- Gas exchange occurs through diffusion, with oxygen moving from alveoli to blood and carbon dioxide moving from blood to alveoli.
Blood Flow to the Lungs at Rest:
- At rest, the lungs receive approximately 4 to 6 liters per minute (L/min) of blood flow.
- This blood flow matches the cardiac output from the right side of the heart.
- However, the pressures and vascular resistance in the pulmonary circulation are different from the systemic circulation.
- The mean pressure in the pulmonary artery is around 15 mmHg, compared to approximately 95 mmHg in the aorta.
- The pressure difference across the pulmonary circulation is not substantial (15 – 5 mmHg).
- Due to this lower pressure difference, resistance in the pulmonary circulation is proportionally lower than in the systemic circulation.
- This is reflected in the anatomy of the pulmonary blood vessels, which are thin-walled and have relatively little smooth muscle.
This information covers pulmonary volumes, pulmonary diffusion, and blood flow to the lungs at rest.
Respiratory Membrane:
- Gas exchange between the air in the alveoli and the blood in the pulmonary capillaries occurs across the respiratory membrane.
- The respiratory membrane is composed of the alveolar wall, the capillary wall, and their respective basement membranes.
- Its primary function is for gas exchange.
- The respiratory membrane is very thin, measuring only 0.5 to 4 mm, allowing gases in the alveoli to be in close proximity to the blood in the capillaries.
Partial Pressures of Gases:
- The air we breathe is a mixture of gases, and each gas exerts a pressure in proportion to its concentration in the mixture, known as partial pressure.
- Dalton’s law states that the total pressure of a gas mixture equals the sum of the partial pressures of the individual gases.
- The air composition at sea level is approximately 79.04% nitrogen (PN2), 20.93% oxygen (PO2), and 0.03% carbon dioxide (PCO2).
- At sea level, the atmospheric pressure (barometric pressure) is about 760 mmHg (standard atmospheric pressure).
- Therefore, PN2 is 600.7 mmHg (79.04% of 760 mmHg), PO2 is 159.1 mmHg (20.93% of 760 mmHg), and PCO2 is 0.2 mmHg (0.03% of 760 mmHg).
- Henry’s law states that gases dissolve in liquids in proportion to their partial pressures, depending on their solubilities and temperature.
- In the human body, gas exchange between alveoli and blood depends primarily on the pressure gradient between the gases in these areas.
This information covers the concept of the respiratory membrane and the partial pressures of gases in the air and their relevance to gas exchange in the body.
Gas Exchange in the Alveoli:
- Differences in partial pressures of gases in the alveoli and blood create a pressure gradient across the respiratory membrane, facilitating gas exchange during pulmonary diffusion.
- Gases move according to partial pressure gradients across the respiratory membrane.
Oxygen Exchange:
- The PO2 of inhaled air at standard atmospheric pressure is 159 mmHg but decreases to about 105 mmHg upon inhalation and mixing with alveolar air.
- Alveolar air is saturated with water vapor and contains more carbon dioxide than inspired air, affecting the total pressure in the alveoli.
- Deoxygenated blood entering pulmonary capillaries has a PO2 of about 40 mmHg, creating a pressure gradient of about 65 mmHg for oxygen exchange.
- As blood moves through the pulmonary capillaries, it equilibrates with alveolar PO2 (105 mmHg), becoming saturated with oxygen.
- The PO2 in the pulmonary vein is 100 mmHg due to some blood being shunted directly to the lung, reducing overall saturation.
Fick’s Law of Diffusion:
- Fick’s law states that the rate of diffusion through a tissue is proportional to surface area, the difference in partial pressure between two sides of the tissue, and inversely proportional to tissue thickness.
- The diffusion constant, unique to each gas, also influences the diffusion rate.
- Oxygen diffusion capacity is about 21 ml/min per 1 mmHg pressure difference at rest, calculated based on the mean partial pressure in the pulmonary capillary.
- During maximal exercise, oxygen diffusion capacity can increase up to three times the resting rate, driven by a greater partial pressure gradient from alveoli to blood.
Blood Flow Changes during Exercise:
- The increase in oxygen diffusion capacity during exercise is attributed to a relatively inefficient circulation at rest, mainly limited perfusion of upper lung regions due to gravity.
- During exercise, blood flow through the lungs increases due to elevated blood pressure, leading to improved lung perfusion.
This information covers gas exchange in the alveoli, oxygen exchange, the principles of Fick’s law of diffusion, and how blood flow changes during exercise to improve lung perfusion.
Carbon Dioxide Exchange:
- Carbon dioxide, like oxygen, moves along a partial pressure gradient.
- Blood passing from the right side of the heart to the alveoli has a PCO2 of about 46 mmHg, while alveolar air has a PCO2 of about 40 mmHg, creating a pressure gradient of about 6 mmHg for CO2 exchange.
- Carbon dioxide’s diffusion coefficient is 20 times greater than that of oxygen, allowing it to diffuse across the respiratory membrane rapidly.
Partial Pressures of Gases Involved in Pulmonary Diffusion:
- Total pressure in venous blood is 706 mmHg, 54 mmHg lower than the total pressure in dry air and alveolar air.
- This difference results from a greater decrease in PO2 compared to the increase in PCO2 as blood passes through the body’s tissues.
Transport of Oxygen and Carbon Dioxide in the Blood:
- Oxygen is transported in the blood mainly by binding to hemoglobin in red blood cells (greater than 98%) or dissolved in plasma (less than 2%).
- Only about 3 ml of oxygen is dissolved in each liter of plasma, which is insufficient to meet the oxygen needs of resting body tissues.
- Hemoglobin allows the blood to transport nearly 70 times more oxygen than can be dissolved in plasma.
This information covers carbon dioxide exchange, the partial pressures of gases involved in pulmonary diffusion, and the transport of oxygen and carbon dioxide in the blood.
Hemoglobin Saturation:
- Over 98% of oxygen is transported in the blood bound to hemoglobin.
- Hemoglobin can carry four molecules of oxygen and forms oxyhemoglobin when oxygen binds to it.
- The oxygen-hemoglobin dissociation curve shows the amount of hemoglobin saturated with oxygen at different PO2 values.
- The curve has a relatively flat upper portion (the “loading” portion) and a steep lower portion (the “unloading” portion).
- In the lungs (high PO2), large drops in PO2 result in only small changes in hemoglobin saturation, encouraging high saturation.
- In the tissues (lower PO2), small changes in PO2 result in large changes in saturation, facilitating oxygen unloading.
- Factors like blood acidity (pH) and temperature affect hemoglobin saturation.
- A decline in pH, seen in the tissues during exercise, shifts the curve to the right (Bohr effect), promoting oxygen unloading.
- Increased blood temperature shifts the curve to the right, causing oxygen to be unloaded more readily at higher temperatures, as in active muscles.
Blood Oxygen-Carrying Capacity:
- The oxygen-carrying capacity of blood primarily depends on its hemoglobin content.
- Each 100 ml of blood contains 14 to 18 g of hemoglobin in men and 12 to 16 g in women.
- Each gram of hemoglobin can combine with about 1.34 ml of oxygen.
- Therefore, the oxygen-carrying capacity of blood is approximately 16 to 24 ml per 100 ml of blood when fully saturated with oxygen.
- Blood remains in contact with alveolar air in the lungs for about 0.75 seconds, allowing hemoglobin to become 98% to 99% saturated during rest.
- People with low hemoglobin concentrations, such as those with anemia, have reduced oxygen-carrying capacities, which can limit their performance during activities with increased oxygen demands.
Carbon Dioxide Transport:
- Carbon dioxide (CO2) is transported in the blood once it’s released from cells.
- It’s carried primarily in three forms: as bicarbonate ions, dissolved in plasma, and bound to hemoglobin (carbaminohemoglobin).
Bicarbonate Ion:
- Bicarbonate ions (HCO3–) account for transporting 60% to 70% of CO2 in the blood.
- CO2 and water combine to form carbonic acid (H2CO3) catalyzed by the enzyme carbonic anhydrase.
- Carbonic acid dissociates into a hydrogen ion (H+) and a bicarbonate ion (HCO3–).
- H+ binds to hemoglobin, triggering the Bohr effect, which shifts the oxygen-hemoglobin dissociation curve to the right.
- Bicarbonate ions diffuse out of red blood cells into plasma, and chloride ions shift into red blood cells to maintain electrical balance (chloride shift).
- Hemoglobin acts as a buffer by binding and neutralizing H+ ions, preventing significant blood acidification.
Dissolved Carbon Dioxide:
- A small amount (7% to 10%) of CO2 is transported in the blood dissolved in plasma.
- Dissolved CO2 comes out of solution where PCO2 is low, such as in the lungs.
- It diffuses from pulmonary capillaries into alveoli and is exhaled.
Carbaminohemoglobin:
- Carbon dioxide can also bind with hemoglobin, forming carbaminohemoglobin.
- Carbaminohemoglobin forms when CO2 binds to amino acids in the globin part of hemoglobin.
- This binding doesn’t compete with oxygen binding because it occurs on a different part of the hemoglobin molecule.
- CO2 binding with hemoglobin depends on oxygenation (deoxyhemoglobin binds CO2 more easily than oxyhemoglobin) and PCO2.
- CO2 is released from hemoglobin in the lungs where PCO2 is low, allowing it to enter alveoli and be exhaled.
Arterial–Venous Oxygen Difference:
- The arterial blood has an oxygen content of about 20 ml of oxygen per 100 ml of blood at rest.
- This decreases to 15 to 16 ml of oxygen per 100 ml in mixed venous blood.
- The difference between arterial and mixed venous oxygen content is called the arterial–mixed venous oxygen difference, or (a-v¯)O2 difference.
- It reflects the 4 to 5 ml of oxygen per 100 ml of blood taken up by tissues.
- The (a-v¯)O2 difference increases with increased oxygen use for oxidative energy production, such as during exercise.
- It can increase to 15 to 16 ml per 100 ml of blood during maximal endurance exercise, and even higher at the local muscle level during intense exercise (17 to 18 ml per 100 ml).
Oxygen Transport in the Muscle:
- Oxygen is transported in the muscle to the mitochondria by myoglobin.
- Myoglobin has a higher affinity for oxygen than hemoglobin.
- Myoglobin releases oxygen only under conditions of very low PO2, which occurs in exercising muscles.
- In the mitochondria of an active muscle, the PO2 can be as low as 1 to 2 mmHg, allowing myoglobin to readily deliver oxygen.
Factors Influencing Oxygen Delivery and Uptake:
- Oxygen delivery and uptake depend on three main variables: oxygen content of blood, blood flow, and local conditions (e.g., pH, temperature).
- A reduction in the blood’s oxygen-carrying capacity or a decrease in arterial PO2 would hinder oxygen delivery and cellular uptake.
- Increased blood flow through muscles during exercise improves oxygen delivery.
- Local changes in muscle during exercise, such as increased acidity due to lactate production, higher temperature, and increased carbon dioxide concentration due to metabolism, facilitate oxygen unloading from hemoglobin and improve oxygen delivery and uptake in the muscles.
Carbon Dioxide Removal:
- Carbon dioxide exits the cells through simple diffusion.
- It moves in response to the partial pressure gradient between the tissue and the capillary blood.
- For example, in muscles, carbon dioxide is generated through oxidative metabolism, leading to a relatively high PCO2 in muscles compared to capillary blood.
- CO2 diffuses out of muscles and into the blood to be transported to the lungs.
Regulation of Pulmonary Ventilation:
- Maintaining homeostatic balance in blood PO2, PCO2, and pH requires coordination between the respiratory and circulatory systems.
- Involuntary regulation of pulmonary ventilation is essential.
- Respiratory muscles are controlled by motor neurons regulated by respiratory centers located within the brain stem (medulla oblongata and pons).
- These centers establish the rate and depth of breathing through impulses to respiratory muscles.
- Voluntary control of respiration can override these centers.
- The inspiratory area (dorsal respiratory group) controls the basic rhythm of ventilation.
- The expiratory area sends signals to expiratory muscles during forceful breathing.
- Other brain centers include the apneustic area and pneumotaxic center, which aid in respiration control.
- Chemical environment changes in the body also influence breathing.
- Central chemoreceptors in the brain respond to an increase in H+ ions in the cerebrospinal fluid, which is influenced by CO2 levels.
- Peripheral chemoreceptors in the aortic arch (aortic bodies) and carotid artery bifurcation (carotid bodies) are sensitive to PO2, H+ concentration, and PCO2.
- PCO2 appears to be the strongest stimulus for regulating breathing, as it influences pH changes.
- Neural mechanisms include stretch receptors in the pleurae, bronchioles, and alveoli, which signal the expiratory center when excessively stretched.
- The Hering-Breuer reflex shortens inspiration duration to prevent overinflating respiratory structures.
- Multiple control mechanisms are involved in regulating breathing to maintain appropriate blood and tissue gas levels and pH for normal cellular function.
- Emotional distress or environmental temperature changes can also affect breathing due to these control mechanisms.